

How Far Does Sound Travel: The Science of Acoustics
Do you ever stop to think about how sound travels? It’s an interesting phenomenon that occurs everyday and yet we often take it for granted. In this blog post, we will explore the science of acoustics and how sound travels. We will answer the question of how far sound can travel and how it is affected by different factors. Stay tuned for an in-depth look at this fascinating topic!

Nature Of Sound
Sound is a mechanical wave that is an oscillation of pressure transmitted through some medium, such as air or water. Sound can propagate through solids and liquids better than gases because the density and stiffness are greater. So how far does sound travel? In this article we will answer how sound travels and how to calculate how far it travels in different scenarios.
Sound Transmits Conception
A common misconception with regard to how sound transmits itself between two points (for example from speaker to ear) is that the source creates waves of compression in the surrounding gas which then proceed on their way at a constant speed until they strike something else; either another solid object or our ears . This analogy might be okay for describing what goes on at low frequencies but once we go beyond around 1000 Hz, the propagation of sound becomes far more complex.

At low frequencies (below around 1000 Hz), sound waves tend to travel in all directions more or less equally and bounce off objects like a rubber ball would. As frequency increases however, the directivity of sound increases as well. So high-frequency sounds are more likely to travel in a straight line between two points than low frequencies. This is why we can often hear someone calling from some distance away when there is loud music playing – because the higher frequencies carry further than the lower ones.
How Far Can Sound Travel
There are three ways that sound can be transmitted: through air, through water, or through solids. The speed of sound through each medium is different and depends on the density and stiffness of the material.

The speed of sound through air is about 343 m/s (or 760 mph), and it travels faster in warmer air than colder air. The speed of sound through water is about 1500 m/s, and it travels faster in salt water than fresh water. The speed of sound through solids is much faster than through either gases or liquids – about 5000-15000 m/s. This is why we can often hear someone coming before we see them – the sound waves are travelling through the solid ground to our ears!
Now that we know how sound propagates and how its speed depends on the medium, let’s take a look at how to calculate how far it will travel between two points. We can use the equation
distance = speed x time
For example, if we want to know how far a sound will travel in one second, we have:
distance = 343 m/s x 0.001 s = 343 m
So sound travels 1 kilometer in roughly 3 seconds and 1 mile in roughly 5 seconds.
Does Вecibel Level affect the Sound Distance?
The surface area around a sound source’s location grows with the square of the distance from the source. This implies that the same amount of sound energy is dispersed over a larger surface, and that the energy intensity decreases as the square of the distance from the source (Inverse Square Law).
Experts of Acoustical control says, that
For every doubling of distance, the sound level reduces by 6 decibels (dB), (e.g. moving from 10 to 20 metres away from a sound source). But the next 6dB reduction means moving from 20 to 40 metres, then from 40 to 80 metres for a further 6dB reduction.
How Far Can Sound Travel In Real World
In real world, there are many factors that can affect how far a sound travels. Factors such as air density, temperature and humidity have an impact on its propagation; obstacles like buildings or mountains could also block some frequencies from going through while letting others pass (this happens because at high frequencies they behave more like waves).
Sounds can propagate through solids better than they can propagate through air because their density/stiffness are greater (this means that sound travels faster). In addition to this, we also know that it takes less time for a high frequency wave to reach us from its source compared with low frequencies. For example if there’s some kind of obstacle blocking our path then it might take longer for waves at higher frequencies than those below 1000 Hz to past them.
Can Sound Waves Travel Infinitely?
No. The higher the frequency of a sound wave, the shorter its wavelength becomes. As wavelength decreases, the amount of energy in a sound wave also decreases and eventually it dissipates completely. This is why we often can’t hear someone calling from very far away when there’s loud music playing – because the high frequencies are being blocked out by all the noise!
Can Sound Travel 20 Miles?
The air may be permeable to these lower-frequency, sub-audible sound waves generated by elephants. Some whale species’ frequencies might travel through seawater for 1500 kilometers or 900 miles.
How Far Can a Human Scream Travel?
The normal intelligible outdoor range of the male human voice in still air is 180 m (590 ft 6.6 in).

The Guiness World Record of the Farthest distance travelled by a human voice belongs the Spanish-speaking inhabitants of the Canary Island of La Gomera, is intelligible under ideal conditions at 8 km (5 miles).
In Conclusion
At the end of this blog post, you should have a better understanding of how sound travel and what factors affect it. If you want to learn more about acoustics and sounds, you can check out our resources here.
- Why PS4 Is So Loud and How to Fix It
- Best Quiet Electric Toothbrush – Buyer’s Guide
You May Also Like
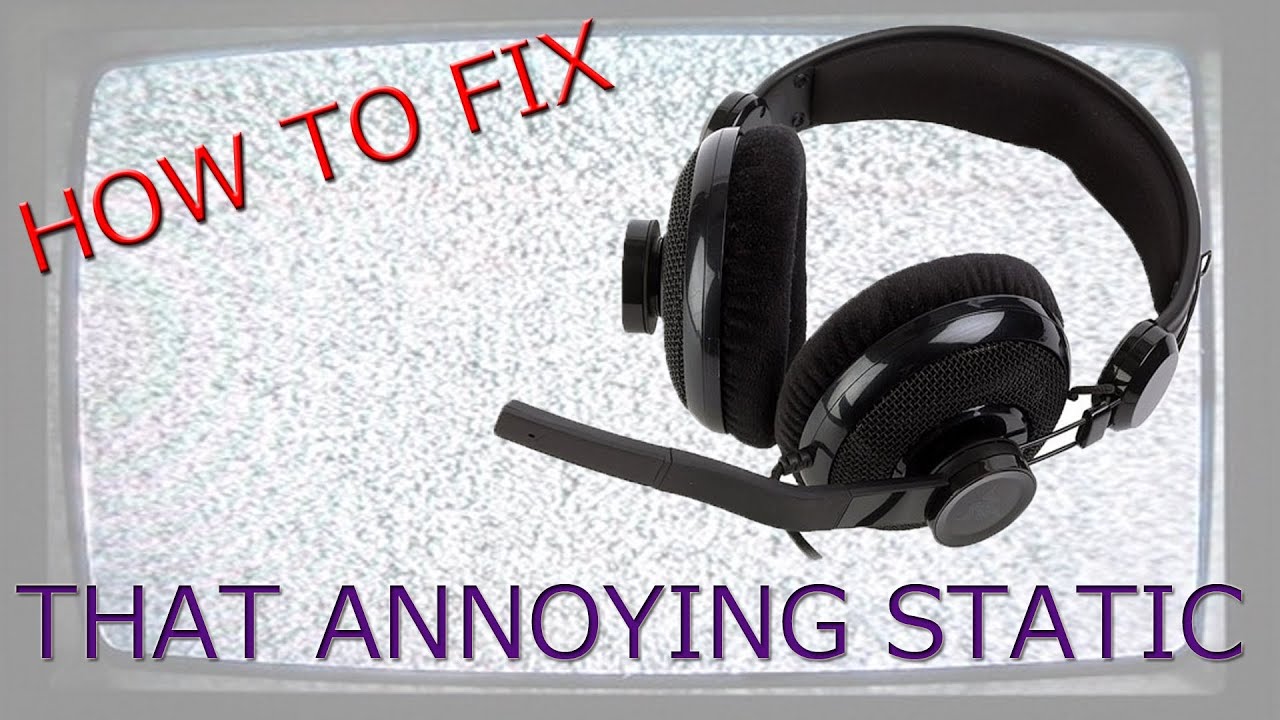
Static Sound in Ear When Loud Noises: What You Need to Know
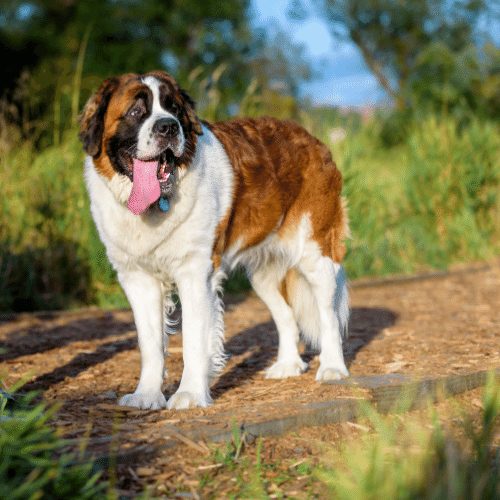
The Quietest Dogs: The Top Ten
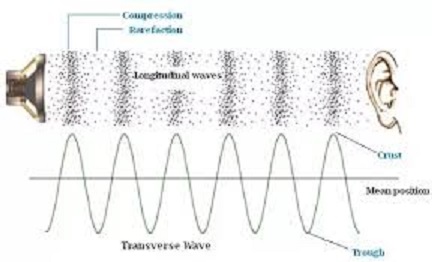
What Direction Does Sound Travel?

Sound Waves: Explanation, Review, and Examples
- The Albert Team
- Last Updated On: September 12, 2023
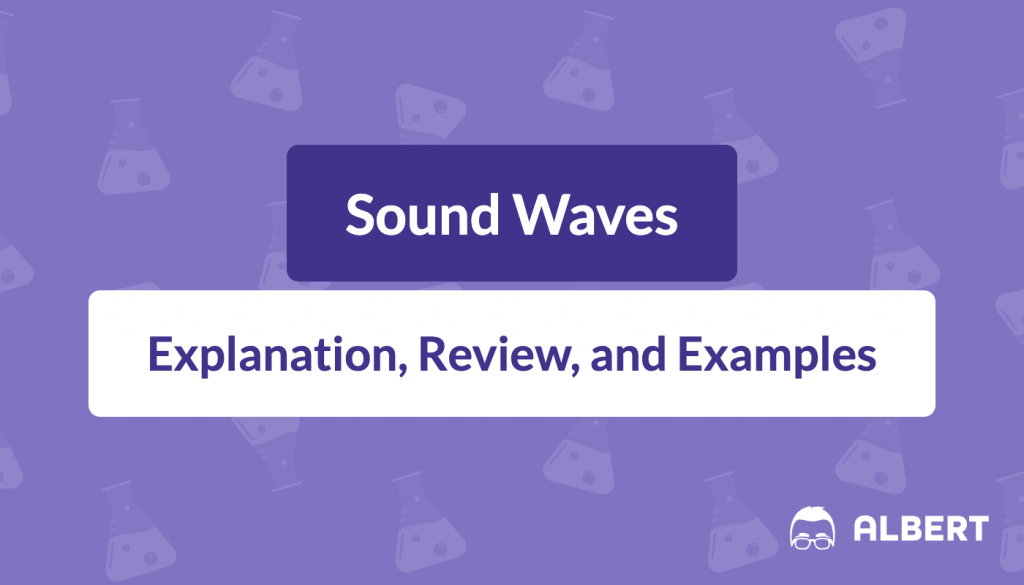
Sound waves fill our world, from music to spoken words to the rustling of leaves in the wind. In this post, we’ll explore what sound waves are, their characteristics like amplitude and frequency, and review the speed of sound. Our aim is to make understanding sound waves straightforward and relatable. So whether you’re curious about how we perceive sound or want to understand the science behind it, this post has got you covered.
What We Review
What Type of Wave is a Sound Wave?
To understand sound, it’s important to know what kind of wave it is. Sound is a longitudinal wave. This means that the particles in the medium (like air) move back and forth in the same direction that the sound wave is traveling. Now let’s break down what this means and why it matters.
Transverse and Longitudinal Waves
Waves can generally be categorized into two types: transverse and longitudinal.
- Transverse Waves: In a transverse wave, the movement of the particles in the medium (could be air, water, etc.) is at right angles to the direction of the wave. Think of a rope being whipped up and down; the wave moves along the length of the rope, but the rope’s actual movement is up and down, perpendicular to the wave direction.
- Longitudinal Waves: In a longitudinal wave, particles in the medium move back and forth along the same line that the wave travels. Imagine pushing and pulling a spring; the compressions and rarefactions (tightening and loosening of the spring’s coils) move along the length of the spring, the same direction in which you pushed or pulled it.
For a full review of transverse and longitudinal waves, be sure to visit our blog post on that topic.
How Sound Waves Are Produced
Sound waves are generated when something vibrates, like a drum skin when struck or vocal cords when you speak. These vibrations push and pull on the air molecules near them, causing these molecules to vibrate back and forth.
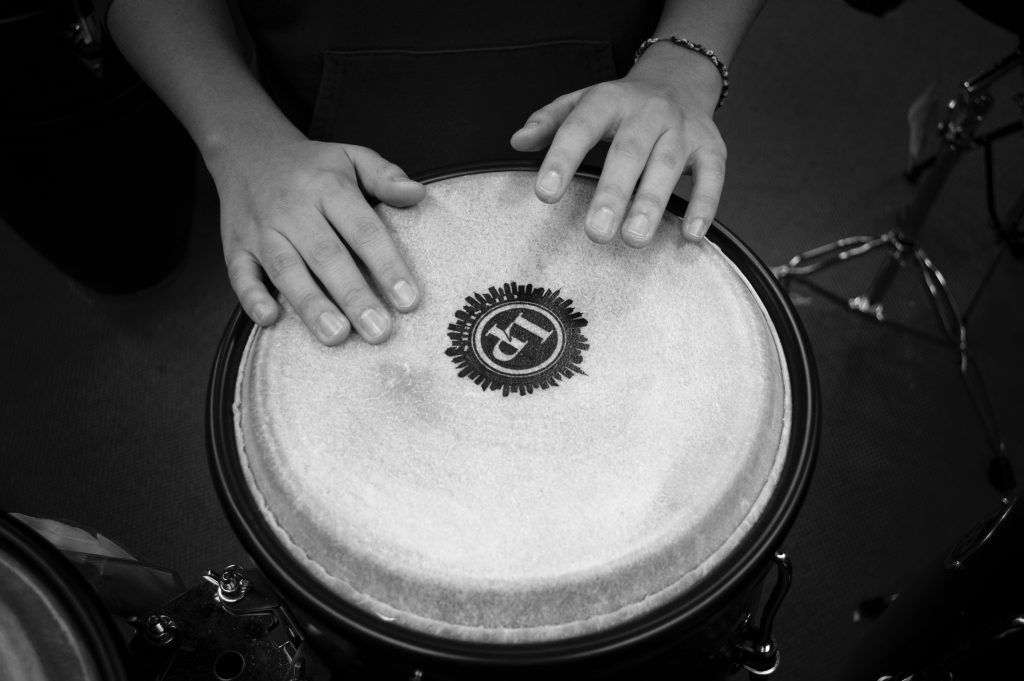
How Sound Waves Travel
Sound, being a longitudinal wave, makes the air molecules move in the same direction as the wave itself. For example, when a drum is hit, the drum’s surface vibrates, causing the nearby air molecules to move back and forth along the line of the wave. This series of compressions (where the air molecules are close together) and rarefactions (where they are spread apart) continue to travel through the air as a sound wave. This is how sound gets from the source (like the drum) to your ears.
Knowing that sound is a longitudinal wave helps you understand how it moves from its source, like a speaker, to your ears. This basic idea is key to understanding more detailed aspects of sound, such as its speed, loudness, and pitch.
Aspects of Sound Waves
Now that we understand sound as a longitudinal wave, we can look at the key features that shape how it behaves. These essential aspects are frequency, amplitude, and speed of sound. If you need to review these basic wave characteristics, visit this post to break down mechanical waves.
Frequency of Sound
Frequency refers to how many times a wave oscillates, or cycles, per second. In terms of sound, frequency determines the pitch of the sound you hear. High-frequency sound waves produce high-pitched noises, like a whistle, while low-frequency sound waves produce low-pitched noises, like a drumbeat. Frequency is measured in hertz (Hz), and in everyday life, human hearing ranges from about 20\text{ Hz} to 20{,}000\text{ Hz} .
Amplitude of Sound
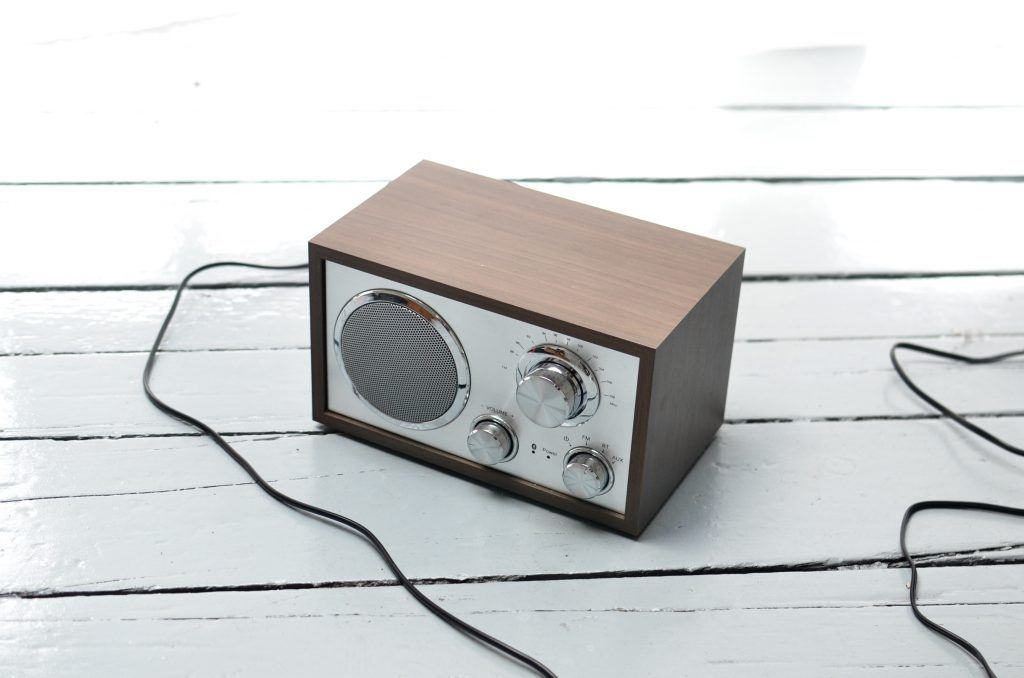
Amplitude is another critical aspect of sound. It describes how “tall” the wave is and is directly related to the loudness of the sound. A sound wave with a higher amplitude will be louder than a sound wave with a lower amplitude. Think of amplitude like the volume knob on your stereo; turning it up increases the amplitude of the sound waves, making the sound louder. Amplitude is typically measured in decibels (dB). The table below shows the sound level in decibels for a range of sources.
Speed of Sound
Lastly, let’s talk about the speed of sound, which varies depending on the medium through which it travels. In dry air at room temperature, the speed of sound is approximately 343\text{ meters per second} . In water, it’s faster—about 1{,}500\text{ meters per second} . The speed of sound is affected by factors like temperature, pressure, and the properties of the medium it’s traveling through.
The table below shows the speed of sound in various media.
By understanding these aspects—frequency, amplitude, and speed—you’ll have a comprehensive view of what sound waves are and how they function. This foundational knowledge sets the stage for a deeper understanding of sound and its many applications in our daily lives.
Technological Applications of Sound Waves
Sound waves have a range of applications in technology, enhancing various aspects of our lives. From musical instruments to medical imaging, the applications are diverse.
Instruments and Music
Musical instruments like guitars, flutes, and drums are classic examples of how sound waves can be manipulated to create harmony and melody. When you pluck a guitar string or hit a drum skin, you’re generating sound waves with specific frequencies and amplitudes. These waves interact to produce the musical tones we enjoy. Understanding the physics behind sound waves helps in the design and optimization of musical instruments, leading to better sound quality and a wider range of tonal possibilities.
Infrasound refers to sound waves with frequencies below the human hearing range, typically less than 20 Hz. Despite being inaudible, these low-frequency waves have several practical applications. They are used in seismology to detect earthquakes and volcanic activity. Infrasound is also used for long-distance communication in certain animal species, such as elephants and whales. Additionally, it has applications in weather forecasting, as some weather phenomena generate infrasonic signals.
Ultrasonic technology
On the opposite end of the spectrum, we have ultrasonic sound waves, which have frequencies higher than the audible range, generally above 20{,}000\text{ Hz} . One of the most well-known applications of ultrasonic waves is in medical imaging, particularly in sonograms and ultrasound scans. These high-frequency waves can penetrate tissues and provide detailed images, making them invaluable in medical diagnostics. Ultrasonic waves are also used in industrial settings for detecting flaws in materials and in distance-measuring devices like sonar.
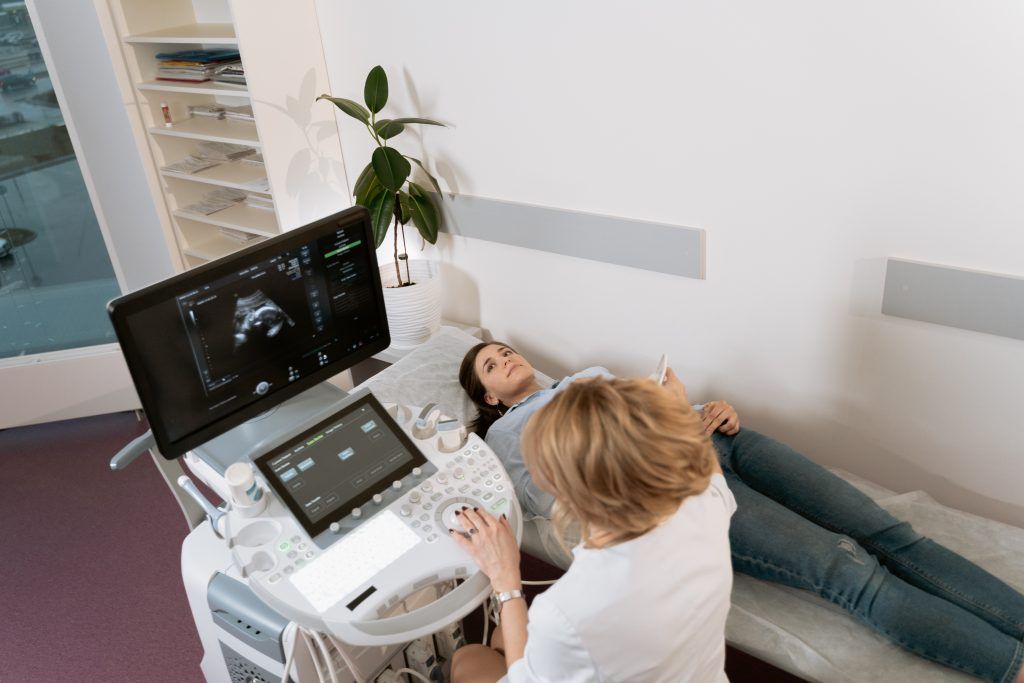
Understanding how sound waves function across different applications not only broadens our appreciation of their versatility but also opens the door for further technological advancements.
In this post, we’ve taken a comprehensive look at sound waves, starting with the basics of what type of wave sound is—a longitudinal wave. We discussed its key aspects like frequency, amplitude, and speed in various media, breaking down how these properties shape our auditory experiences. Then, we explored the practical applications of sound waves in technology, focusing on musical instruments, infrasound, and ultrasonics. Whether you’re interested in how we hear everyday sounds or in the broader technological applications, understanding these fundamentals provides a solid foundation for further exploration into the world of sound.
Interested in a school license?
Popular posts.
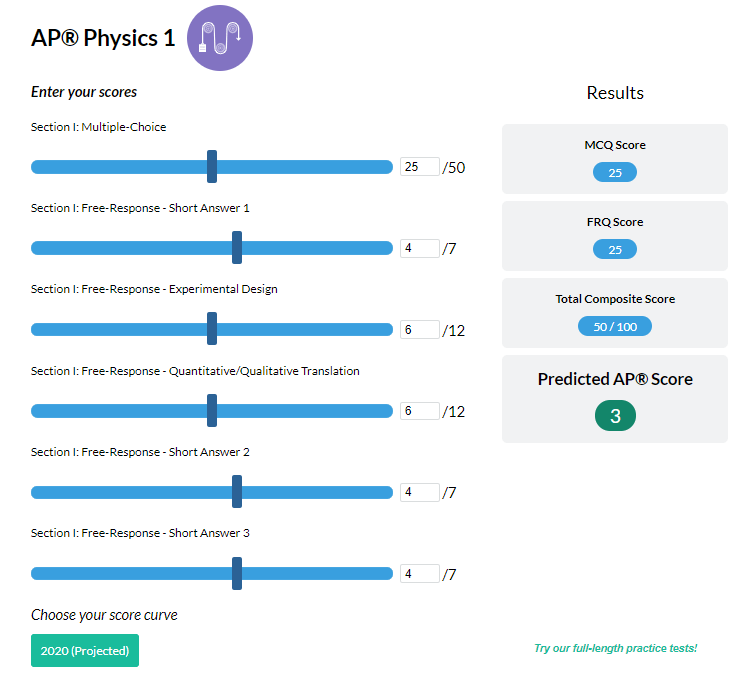
AP® Score Calculators
Simulate how different MCQ and FRQ scores translate into AP® scores
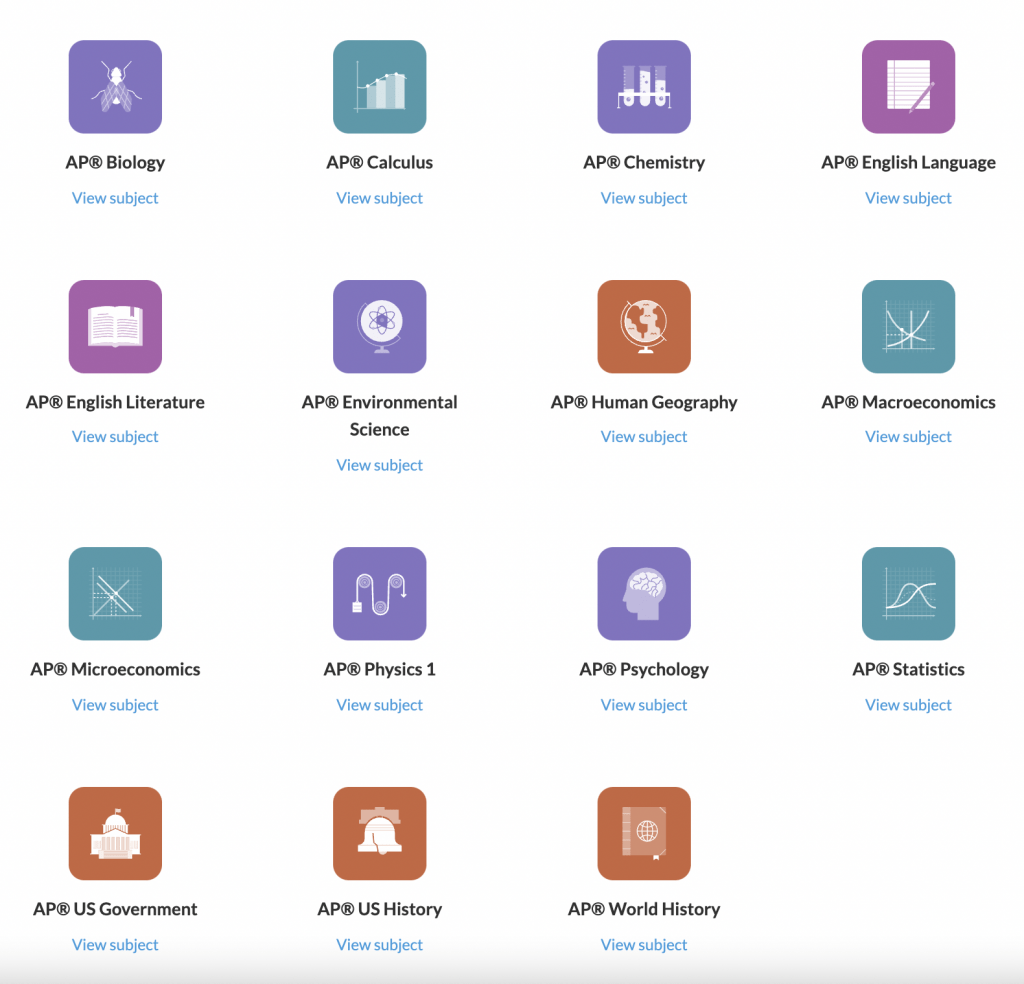
AP® Review Guides
The ultimate review guides for AP® subjects to help you plan and structure your prep.
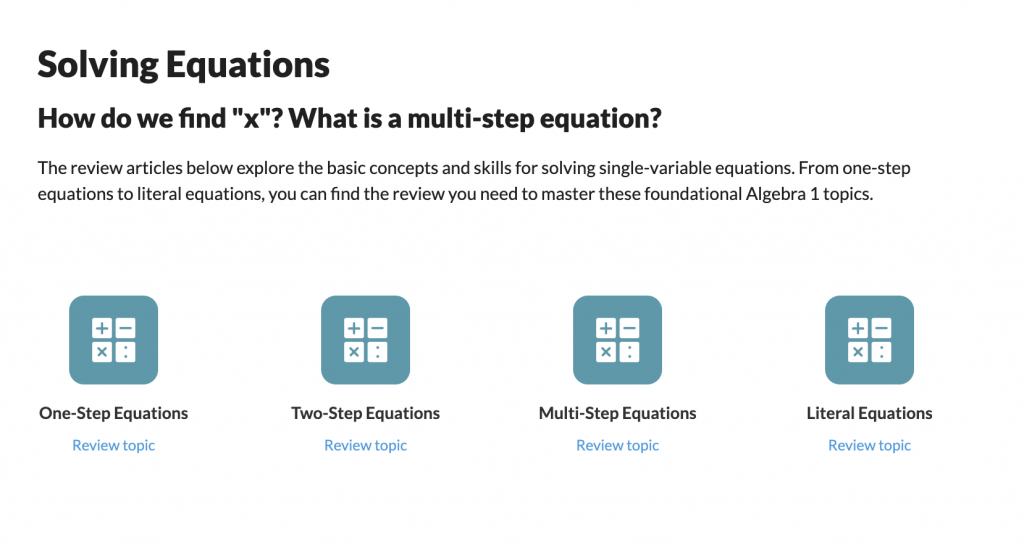
Core Subject Review Guides
Review the most important topics in Physics and Algebra 1 .
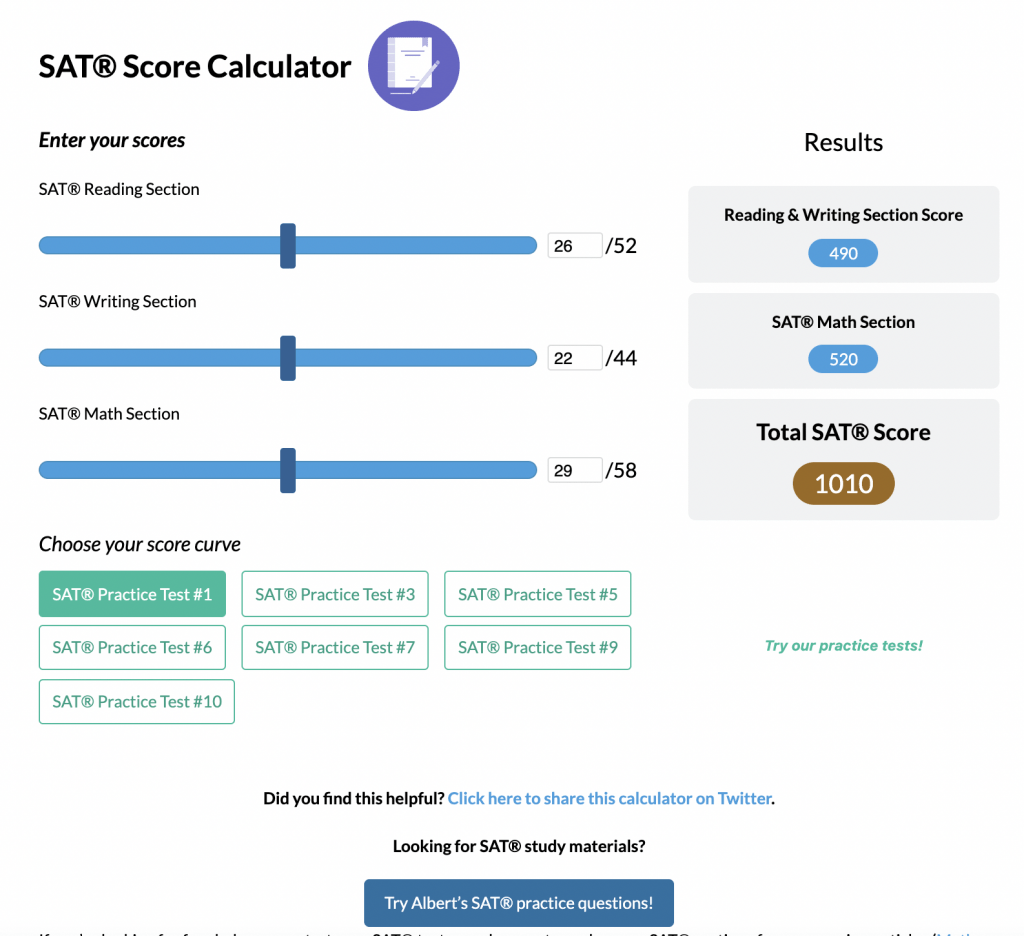
SAT® Score Calculator
See how scores on each section impacts your overall SAT® score
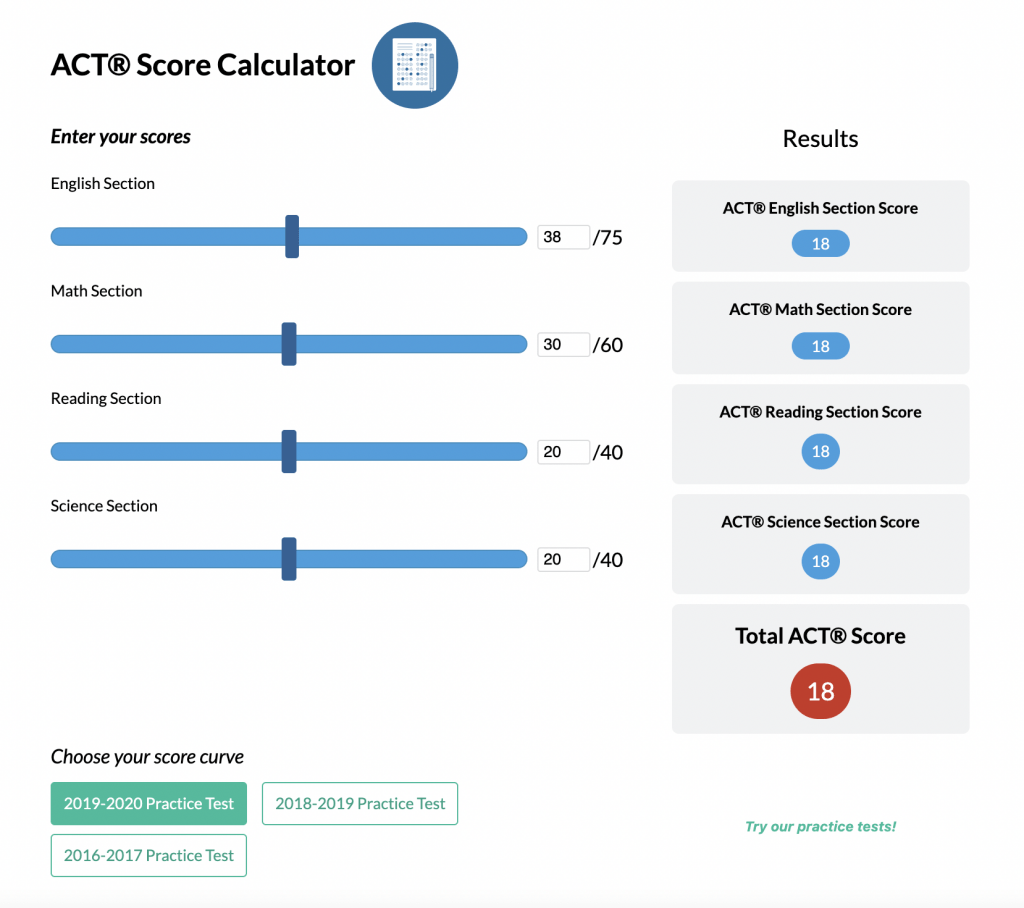
ACT® Score Calculator
See how scores on each section impacts your overall ACT® score
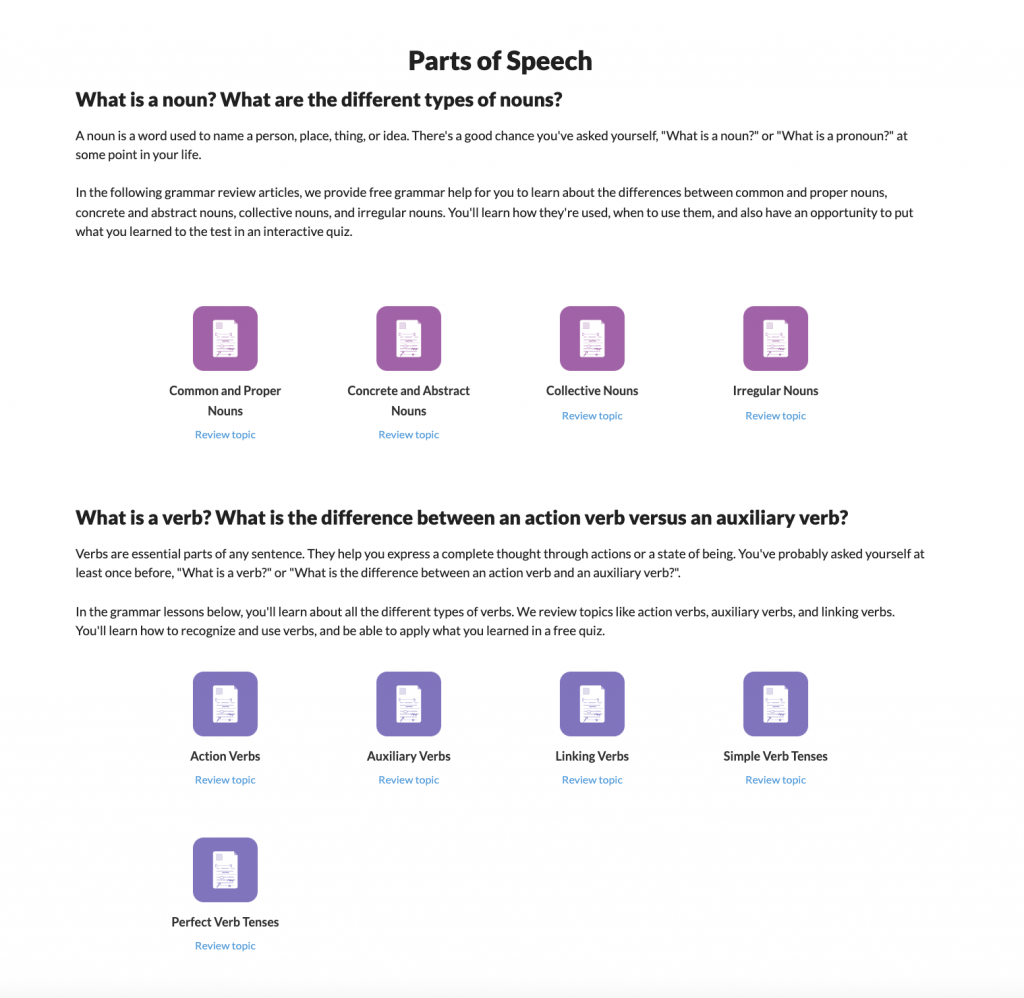
Grammar Review Hub
Comprehensive review of grammar skills
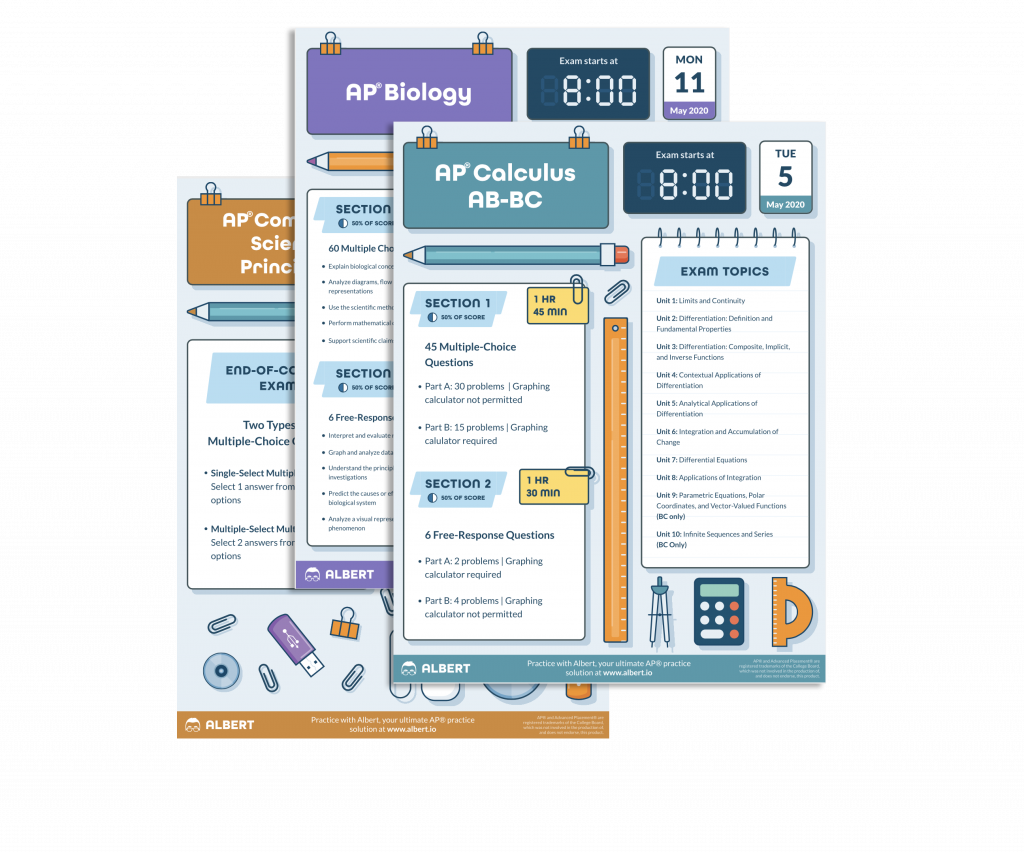
AP® Posters
Download updated posters summarizing the main topics and structure for each AP® exam.

Advertisement
Understanding Sound Waves and How They Work
- Share Content on Facebook
- Share Content on LinkedIn
- Share Content on Flipboard
- Share Content on Reddit
- Share Content via Email
Sound. When a drum is struck, the drumhead vibrates and the vibrations are transmitted through the air in the form of sound waves . When they strike the ear, these waves produce the sensation of sound.
Technically, sound is defined as a mechanical disturbance traveling through an elastic medium — a material that tends to return to its original condition after being deformed. The medium doesn't have to be air. Metal, wood, stone, glass, water, and many other substances conduct sound — many of them even better than air.
The Basics of Sound
Sound waves, speed of sound, the behavior of a sound wave, sound quality, history of sound.
There are many sources of sound. Familiar kinds include the vibration of a person's vocal cords, vibrating strings (piano, violin), a vibrating column of air (trumpet, flute), and vibrating solids (a door when someone knocks). It's impossible to list them all because anything that imparts a disturbance to an elastic medium is a source of sound.
Sound can be described in terms of pitch — from the low rumble of distant thunder to the high-pitched buzzing of a mosquito — and loudness. Pitch and loudness , however, are subjective qualities; they depend in part on the hearer's sense of hearing. Objective, measurable qualities of sound include frequency and intensity, which are related to pitch and loudness. These terms, as well as others used in discussing sound, are best understood through an examination of sound waves and their behavior.
Speed of sound in various mediums
Air, like all matter, consists of molecules. Even a tiny region of air contains vast numbers of air molecules. The molecules are in constant motion, traveling randomly and at great speed. They constantly collide with and rebound from one another and strike and rebound from objects that are in contact with the air.
When an object vibrates it produces sound waves in the air. For example, when the head of a drum is hit with a mallet, the drumhead vibrates and produces sound waves. The vibrating drumhead produces sound waves because it moves alternately outward and inward, pushing against, then moving away from, the air next to it. The air particles that strike the drumhead while it is moving outward rebound from it with more than their normal energy and speed, having received a push from the drumhead.
These faster-moving molecules move into the surrounding air. For a moment, the region next to the drumhead has a greater-than-normal concentration of air molecules — it becomes a region of compression. As the faster-moving molecules overtake the air molecules in the surrounding air, they collide with them and pass on their extra energy. The region of compression moves outward as the energy from the vibrating drumhead is transferred to groups of molecules farther and farther away.
Air molecules that strike the drumhead while it's moving inward rebound from it with less than their normal energy and speed. For a moment, the region next to the drumhead has fewer air molecules than normal — it becomes a region of rarefaction. Molecules colliding with these slower-moving molecules also rebound with less speed than normal, and the region of rarefaction travels outward.
The nature of sound is captured through its fundamental characteristics : wavelength (the distance between wave peaks), amplitude (the height of the wave, corresponding to loudness), frequency (the number of waves passing a point per second, related to pitch), time period (the time it takes for one complete wave cycle to occur), and velocity (the speed at which the wave travels through a medium). These properties intertwine to craft the unique signature of every sound we hear.
The wave nature of sound becomes apparent when a graph is drawn to show the changes in the concentration of air molecules at some point as the alternating pulses of compression and rarefaction pass that point. The graph for a single pure tone, such as that produced by a vibrating tuning fork, would show a sine wave (illustrated here ). The curve shows the changes in concentration. It begins, arbitrarily, at some time when the concentration is normal and a compression pulse is just arriving. The distance of each point on the curve from the horizontal axis indicates how much the concentration varies from normal.
Each compression and the following rarefaction make up one cycle. (A cycle can also be measured from any point on the curve to the next corresponding point.) The frequency of a sound is measured in cycles per second or hertz (abbreviated Hz). The amplitude is the greatest amount by which the concentration of air molecules varies from the normal.
The wavelength of a sound is the distance the disturbance travels during one cycle. It's related to the sound's speed and frequency by the formula speed/frequency = wavelength. This means that high-frequency sounds have short wavelengths and low-frequency sounds have long wavelengths. The human ear can detect sounds with frequencies as low as 20 Hz and as high as 20,000 Hz. In still air at room temperature, sounds with these frequencies have wavelengths of 75 feet (23 m) and 0.68 inch (1.7 cm) respectively.
Intensity refers to the amount of energy transmitted by the disturbance. It's proportional to the square of the amplitude. Intensity is measured in watts per square centimeter or in decibels (db). The decibel scale is defined as follows: An intensity of 10-16 watts per square centimeter equals 0 db. (Written out in decimal form, 10-16 appears as 0.0000000000000001.) Each tenfold increase in watts per square centimeter means an increase of 10 db. Thus, an intensity of 10-15 watts per square centimeter can also be expressed as 10 db and an intensity of 10-4 (or 0.0001) watts per square centimeter as 120 db.
The intensity of sound drops rapidly with increasing distance from the source. For a small sound source radiating energy uniformly in all directions, intensity varies inversely with the square of the distance from the source. That is, at a distance of two feet from the source the intensity is one-fourth as great as it is at a distance of one foot; at three feet it is only one-ninth as great as at one foot, etc.
Pitch depends on the frequency ; in general, a rise in frequency causes a sensation of rising pitch. The ability to distinguish between two sounds that are close in frequency, however, decreases in the upper and lower parts of the audible frequency range. There is also variation from person to person in the ability to distinguish between two sounds of very nearly the same frequency. Some trained musicians can detect differences in frequency as small as 1 or 2 Hz.
Because of how the hearing mechanism functions, the perception of pitch is also affected by intensity. Thus, when a tuning fork vibrating at 440 Hz (the frequency of A above middle C on the piano) is brought closer to the ear, a slightly lower tone, as though the fork were vibrating more slowly, is heard.
When the source of a sound is moving at a relatively high speed, a stationary listener hears a sound higher in pitch when the source is moving toward him or her and a sound lower in pitch when the source is moving away. This phenomenon, known as the Doppler effect , is due to the wave nature of sound.
In general, an increase in intensity will cause a sensation of increased loudness. But loudness does not increase in direct proportion to intensity. A sound of 50 dB has ten times the intensity of a sound of 40 dB but is only twice as loud. Loudness doubles with each increase of 10 dB in intensity.
Loudness is also affected by frequency because the human ear is more sensitive to some frequencies than to others. The threshold of hearing — the lowest sound intensity that will produce the sensation of hearing for most people — is about 0 dB in the 2,000 to 5,000 Hz frequency range. For frequencies below and above this range, sounds must have greater intensity to be heard. Thus, for example, a sound of 100 Hz is barely audible at 30 dB; a sound of 10,000 Hz is barely audible at 20 dB. At 120 to 140 dB, most people experience physical discomfort or actual pain, and this level of intensity is referred to as the threshold of pain .
When we visualize waves, we often think of transverse waves — like the rolling waves on a beach — where the motion of the wave is perpendicular to the direction of energy transfer. However, sound waves are a different type altogether — a longitudinal wave. In longitudinal sound waves, such as sound waves produced by a vibrating drumhead or our vocal cords, the particles of the medium move parallel to the wave's direction of travel. This movement creates areas of compression and rarefaction in the medium — be it air, water, or a solid — which our ears interpret as sound. Understanding the difference between longitudinal and transverse waves is central to understanding sound.
The speed of sound depends on the elasticity and density of the medium through which it is traveling. In general, sound travels faster in liquids than in gases and faster in solids than in liquids. The greater the elasticity and the lower the density, the faster sound moves in a medium. The mathematical relationship is speed = (elasticity/density).
The effect of elasticity and density on the speed of sound can be seen by comparing the speed of sound in air, hydrogen, and iron. Air and hydrogen have nearly the same elastic properties, but the density of hydrogen is less than that of air. Sound travels faster (about 4 times as fast) in hydrogen than in air. Although the density of air is much less than that of iron, the elasticity of iron is very much greater than that of air. Sound travels faster (about 14 times as fast) in iron than in air.
The speed of sound in a material, particularly in a gas or liquid, varies with temperature because a change in temperature affects the material's density. In air, for example, the speed of sound increases with an increase in temperature . At 32 °F. (0 °C.), the speed of sound in air is 1,087 feet per second (331 m/s); at 68 °F. (20 °C.), it is 1,127 feet per second (343 m/s).
The terms subsonic and supersonic refer to the speed of an object, such as an airplane, in relation to the speed of sound in the surrounding air. A subsonic speed is below the speed of sound; a supersonic speed is above the speed of sound. An object traveling at supersonic speed produces shock waves rather than ordinary sound waves. A shock wave is a compression wave that, when produced in air, can usually be heard as a sonic boom .
The speeds of supersonic objects are often expressed in terms of Mach number — the ratio of the object's speed to the speed of sound in the surrounding air. Thus, an object traveling at Mach 1 is traveling at the speed of sound; at Mach 2, it is traveling at twice the speed of sound.
Like light waves and other waves, sound waves are reflected, refracted, and diffracted, and exhibit interference.
Sound is constantly being reflected off many different surfaces. Most of the time the reflected sound is not noticed, because two identical sounds that reach the human ear less than 1/15 of a second apart cannot be distinguished as separate sounds. When the reflected sound is heard separately, it's called an echo .
Sound is reflected from a surface at the same angle at which it strikes the surface. This fact makes it possible to focus sound by means of curved reflecting surfaces in the same way that curved mirrors can be used to focus light. It also accounts for the effects of so-called whispering galleries, rooms in which a word whispered at one point can be heard distinctly at some other point fairly far away, though it cannot be heard anywhere else in the room. (The National Statuary Hall of the United States Capitol is an example.) Reflection is also used to focus sound in a megaphone and when calling through cupped hands.
The reflection of sound can pose a serious problem in concert halls and auditoriums. In a poorly designed hall, a speaker's first word may reverberate (echo repeatedly) for several seconds, so that the listeners may hear all the words of a sentence echoing at the same time. Music can be similarly distorted. Such problems can usually be corrected by covering reflecting surfaces with sound-absorbing materials such as draperies or acoustical tiles. Clothing also absorbs sound; for this reason, reverberation is greater in an empty hall than in one filled with people. All these sound-absorbing materials are porous; sound waves entering the tiny air-filled spaces bounce around in them until their energy is spent. They are, in effect, trapped.
The reflection of sound is used by some animals, notably bats , for echolocation — locating, and in some cases identifying, objects through the sense of hearing rather than the sense of sight. Bats emit bursts of sound of frequencies far beyond the upper limits of human hearing. Sounds with short wavelengths are reflected even from very small objects. A bat can unerringly locate and catch even a mosquito in total darkness. Sonar is an artificial form of echolocation .
When a wave passes from one material to another at an angle, it usually changes speed, causing the wave front to bend. The refraction of sound can be demonstrated in a physics laboratory by using a lens-shaped balloon filled with carbon dioxide to bring sound waves to a focus.
Diffraction
When sound waves pass around an obstacle or through an opening in an obstacle, the edge of the obstacle or the opening acts as a secondary sound source, sending out waves of the same frequency and wavelength (but of lower intensity) as the original source. The spreading out of sound waves from the secondary source is called diffraction . Because of this phenomenon, sound can be heard around corners despite the fact that sound waves generally travel in a straight line.
Interference
Whenever waves interact, interference occurs. For sound waves, the phenomenon is perhaps best understood by thinking in terms of the compressions and rarefactions of the two waves as they arrive at some point. When the waves are in phase so that their compressions and rarefactions coincide, they reinforce each other ( constructive interference ). When they are out of phase, so that the compressions of one coincide with the rarefactions of the other, they tend to weaken or even cancel each other ( destructive interference ). The interaction between the two waves produces a resultant wave.
In auditoriums, destructive interference between sound from the stage and sound reflected from other parts of the hall can create dead spots in which both the volume and clarity of sound are poor. Such interference can be reduced by the use of sound-absorbing materials on reflecting surfaces. On the other hand, interference can improve an auditorium's acoustical qualities. This is done by arranging the reflecting surfaces in such a way that the level of sound is actually increased in the area in which the audience sits.
Interference between two waves of nearly but not quite equal frequencies produces a tone of alternately increasing and decreasing intensity because the two waves continually fall in and out of phase. The pulsations heard are called beats. Piano tuners make use of this effect, adjusting the tone of a string against that of a standard tuning fork until beats can no longer be heard.
Sound waves are fundamentally pressure waves, traveling through the compression and rarefaction of particles within a medium. Sound waves consist of areas where particles are bunched together, followed by areas where they're spread apart. These high-pressure and low-pressure regions propagate through environments such as air, water or solids, as the energy of the sound wave moves from particle to particle. It's the rapid variation in pressure that an ear drum detects and the brain decodes into the sounds we hear.
Sounds of a single pure frequency are produced only by tuning forks and electronic devices called oscillators ; most sounds are a mixture of tones of different frequencies and amplitudes. The tones produced by musical instruments have one important characteristic in common: they are periodic, that is, the vibrations occur in a repeating pattern. The oscilloscope trace of a trumpet's sound shows such a pattern. For most non-musical sounds, such as those of a bursting balloon or a person coughing, an oscilloscope trace would show a jagged, irregular pattern, indicating a jumble of frequencies and amplitudes.
A column of air, as that in a trumpet, and a piano string both have a fundamental frequency — the frequency at which they vibrate most readily when set in motion. For a vibrating column of air, that frequency is determined principally by the length of the column. (The trumpet's valves are used to change the effective length of the column.) For a vibrating string, the fundamental frequency depends on the string's length, its tension, and its mass per unit length.
In addition to its fundamental frequency, a string or vibrating column of air also produces overtones with frequencies that are whole-number multiples of the fundamental frequency. It is the number of overtones produced and their relative strength that gives a musical tone from a given source its distinctive quality or timbre . The addition of further overtones would produce a complicated pattern, such as that of the oscilloscope trace of the trumpet's sound.
How the fundamental frequency of a vibrating string depends on the string's length, tension, and mass per unit length is described by three laws:
1. The fundamental frequency of a vibrating string is inversely proportional to its length.
Reducing the length of a vibrating string by one-half will double its frequency, raising the pitch by one octave, if the tension remains the same.
2. The fundamental frequency of a vibrating string is directly proportional to the square root of the tension.
Increasing the tension of a vibrating string raises the frequency; if the tension is made four times as great, the frequency is doubled, and the pitch is raised by one octave.
3. The fundamental frequency of a vibrating string is inversely proportional to the square root of the mass per unit length.
This means that of two strings of the same material and with the same length and tension, the thicker string has the lower fundamental frequency. If the mass per unit length of one string is four times that of the other, the thicker string has a fundamental frequency one-half that of the thinner string and produces a tone one octave lower.
One of the first discoveries regarding sound was made in the sixth century B.C. by the Greek mathematician and philosopher Pythagoras . He noted the relationship between the length of a vibrating string and the tone it produces — what is now known as the first law of strings. Pythagoras may also have understood that the sensation of sound is caused by vibrations. Not long after his time it was recognized that this sensation depends on vibrations traveling through the air and striking the eardrum.
About 1640 the French mathematician Marin Mersenne conducted the first experiments to determine the speed of sound in air. Mersenne is also credited with discovering the second and third laws of strings. In 1660 the British scientist Robert Boyle demonstrated that the transmission of sound required a medium — by showing that the ringing of a bell in a jar from which the air had been pumped could not be heard.
Ernst Chladni , a German physicist, made extensive analyses of sound vibrations during the late 1700s and early 1800s. In the early 1800s, the French mathematician Fourier discovered that such complex waves as those produced by a vibrating string with all its overtones consist of a series of simple periodic waves.
An important contribution to the understanding of acoustics was made by Wallace Clement Sabine , a physicist at Harvard University, in the late 1890s. Sabine was asked to improve the acoustics of the main lecture hall in Harvard's Fogg Art Museum. He was first to measure reverberation time — which he found to be 5 1/2 seconds in the lecture hall. Experimenting first with seat cushions from a nearby theater, and later with other sound-absorbing materials and other methods, Sabine laid the foundation for architectural acoustics. He designed Boston Symphony Hall (opened in 1900), the first building with scientifically formulated acoustics.
In the second half of the 20th century, the rising level of noise in the modern world — particularly in urban areas — prompted a whole new series of investigations, dealing in large part with the physiological and psychological effects of noise on humans.
This article was updated in conjunction with AI technology, then fact-checked and edited by a HowStuffWorks editor.
Please copy/paste the following text to properly cite this HowStuffWorks.com article:
- Skip to primary navigation
- Skip to main content
- Skip to footer
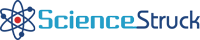
Science Struck
How Does Sound Travel? Here’s the Science Behind This Concept
When sound waves travel through a medium, the particles of the medium vibrate. Vibrations reach the ear and then the brain which senses them and we recognize sound. Read on for an explanation of how sound travels.
Like it? Share it!
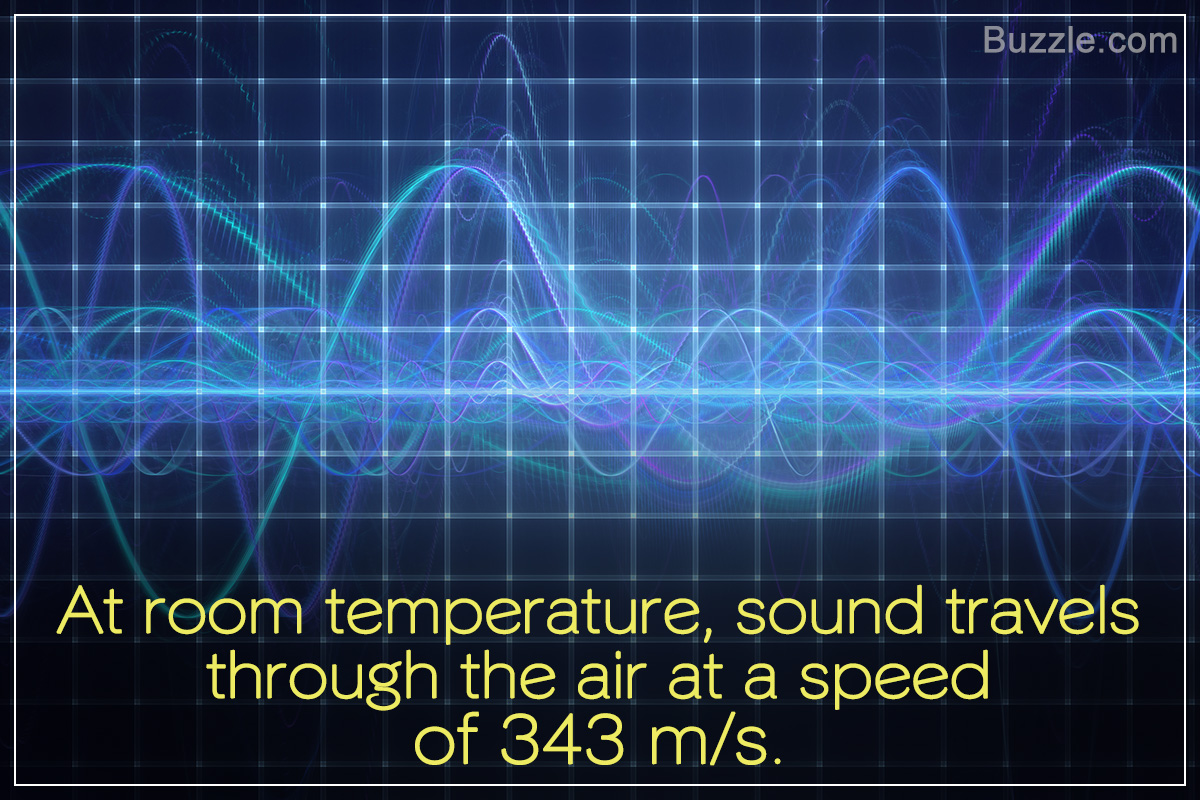
Sound is a series of compression and rarefraction waves that can travel long distances. It is produced by the vibration of the particles present in its medium; a medium is the material through which sound can travel. Presence of a medium is a must for the movement of sound waves. There are various types of medium through which sound waves can move like solids, liquids, gases, plasma, etc. Sound cannot travel through vacuum.
Characteristics of Sound Waves
The speed and the physical characteristics of sound largely varies with the change in its ambient conditions. The speed of sound depends on the density of the medium though which it is traveling. If its density is quite high, then sound would travel at a faster pace. When sound travels through gaseous medium, its speed varies with respect to changes in temperature.
The frequency of sound waves is nothing but the total number of vibrations that have been produced. The length of sound waves vary according to its frequency. Sound waves with long wavelengths have low frequency or low pitch; and those with short wavelengths have high frequency or high pitch. Our ears are capable of hearing only those sound waves which lie in the range between 20 and 20,000 vibrations per second.
How do Sound Waves Travel?
Basically, there are three things that are required for the transmission of sound. They are: a source that can transmit the sound, a medium through which sound can pass (like, water, air, etc.), and the receiver or the detector which receives the sound. The traveling process of sound has been explained below.
Creation of Sound
When a physical object moves in air, it causes vibrations which leads to formation of a series of compression waves in the air. These waves travel in the form of sound. For instance, when we strum the strings of a guitar or hit the head of a drum, the to-and-fro motion of the strings or the drum head creates compression waves of sound in the surrounding air. Similarly, when we speak, our vocal cords vibrate and the sound is created. This type of vibration occurs not just in atmospheric air but in other mediums like, solids and liquids as well. For instance, when a train is moving on a railroad made up of steel, the sound waves thus produced travel via these tracks.
At room temperature, sound travels through air with a speed of 343 m/s, through water at 1,482 m/s, and through steel at 5,960 m/s. As you can see, sound waves travel in a gaseous medium at a slow pace because its molecules are loosely bound and have to cover a long distance to collide with another molecule. In solid medium, the atoms are so closely packed that the vibration is readily transmitted to the neighboring atoms, and sound travels quite fast. In liquid medium, the bonding between the component particles are not as strong as in solids. Therefore, the sound waves move through it at a less speed as compared to solid.
Detection of Sound
When the sound waves hit the receiver, it causes some vibration in that object. The detector captures just a part of the energy from the moving sound wave. This energy of vibration is then converted to electrical signals. Thus, when the sound waves reach our ears, the eardrum present inside it vibrates. This vibration reaches our inner ear and is converted into nerve signals. As a result, we can hear the sound. Devices like microphone can detect sound. The sound waves create vibrations in its membrane which forms electrical signals that gets amplified and recorded.
So, how does sound travel? Vibration of an object causes vibrations of the same frequency in the surrounding medium. The vibrations are sent to the inner ear. After the auditory nerve picks up these vibrations, electrical signals are sent to the brain where the vibrations are recognized as sound.
Get Updates Right to Your Inbox
Privacy overview.
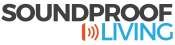
Does Sound Travel Up or Down in an Apartment?
I’ve often found that the biggest threat to soundproofing solutions in apartment buildings comes from shared walls. But does that mean that you should neglect the floors and ceilings? Today, we’re going to settle the question once and for all. So let’s figure out whether sound travels up or down in an apartment building .
Well, while many people think that sound travels in one direction, that’s actually just a misconception . People imagine the waves of music that leave their speakers as going out in a straight line or in a conical shape from the speaker. But in order to have sound travel toward a particular area, you’d have to physically direct it . The best way to do that is to use something like a tube or a cone — which is why we have megaphones.

Still, most people aren’t directing the noise they make in their own home. They’re just walking around their apartments, watching movies, and living their lives as usual. So where does all that sound go? Well, if we want to understand the direction of sound, we first need to know how it’s dispersed .
How Sound Travels
Understanding the various types of noises is the first step to being able to defend against them. That’s why I always recommend reading my article on impact and airborne noise before embarking on your first soundproofing project.
You see, when you’re trying to figure out where noise goes after leaving its source, you have to keep in mind its mode of transportation as well. How sound travels is a much more important question here.
Imagine, if you will, setting up a speaker system on the floor of your apartment and playing your music with the bass turned down low. If your neighbors could hear it, that would mean that the noise was traveling through the air. As scores of science fiction movies keep telling us, sound can’t exist in a vacuum. So no air, no sound .
If I were to recommend a soundproofing solution to prevent this type of noise from seeping out of your apartment, my first suggestion would be to plug all of the holes in walls, ceilings, and floors . However, there is an even more persistent type of noise.
If you were to turn up the bass dial on your speaker, you might start to feel the floor shake a little bit . Because this type of sound travels through the building structure, we usually call it structure-borne noise — or impact noise . This is why you’re particularly annoyed when your upstairs neighbor keeps stomping above your head, and you can feel the building trembling because a bus just shuffled past it.
Although most airborne noise isn’t able to carry through solid floors and ceilings, lower bass frequencies might. Ultimately, floors and ceilings are thicker and denser than walls, so they won’t let the higher frequency sounds through as easily .

The Positioning of the Source of Sound
The other important factor to keep in mind when thinking about the direction of sound is the positioning of the device that’s making it. If you were looking to lessen the amount of noise that passes through your walls, whether through the air or the structure of the building, you may want to move the source of the sound away from more vulnerable areas. That’s why I always say to move the TV or the computer away from shared walls .
However, positioning also matters in the question we’re trying to answer today. And since gravity compels us to have all of the noise-makers in our homes connect to the floor, you could say that sound travels downward.
Let’s take speakers as an example again. If you have your speakers sitting directly on the desk, they will vibrate against it. The sound will carry through the structure of the desk, into the floor, and to the neighbors below.
However, your neighbor is unlikely to complain about this type of noise. Your footsteps are what you really should be worried about, no matter how light on your feet you think you are. If you want to make sure that you’re not bothering your downstairs neighbors, you could try out some of my floor soundproofing tips .
So in this case, it’s safe to say that noise is more likely to travel down . When is the last time you heard sounds coming from the apartment below? It’s rare, but it happens. The sound could carry up if there were fewer obstructions in the way . So you might hear something if both your windows and your downstairs neighbors’ windows were open. Impact noise could also travel up, as in the case of a passing bus.
So Does Sound Travel Up or Down in an Apartment?
The answer is as complex as the subject matter. Technically, there are certain ways to change your environment in order to point sound toward a particular area.
Generally, though, sound travels in all directions. After all, you hear your speakers even if they’re facing away from you, right? But even though that’s technically the case, you’re probably hearing more noise coming from upstairs than downstairs. Fortunately, you can fix that by implementing some simple soundproofing techniques.
RELATED POSTS:
- How To Soundproof Any Floor
- Best Acoustic Floor Underlayment
- How To Soundproof A Ceiling
6 thoughts on “Does Sound Travel Up or Down in an Apartment?”
How can I locate where the sound comes from: upstairs or downstairs appartment? The sound we are hearing is an engine when it is on, like a generator. And what a relief when it is turned off! Unfortunately the upstairs neighbors will not allow any acoustic engineer to verify it and suggest a solution.
Hi Lucas! Start by walking around your apartment to pinpoint where the sound is the loudest. If it’s louder near the ceiling, it’s likely coming from upstairs and if it’s louder near the floor, it could be from downstairs. You can also use a simple tube to help focus the sound and better identify its direction.
This is an interesting phenomenon. I live on the bottom floor in an apartment that has (3) apartments above mine within the same “footprint” of the building. The sound of the shoes, feet what-have-you, is super, super loud. Often I’m given a terrible “start” with accompanied heart dropping to my feet. Imagine a bowling ball being dropped and landing on a floor/ceiling less than 3′ from your ears. Its a shock ! Any time the neighbors simply travel across their apartment it is very much like a herd. Yet, in the case of the most constant offenders, I have never heard a peep ! This is consistent throughout the entire apartment.
You’re 100 percent right. I try to tell my upstairs neighbors that the footsteps are loud and travel down into my apartment, so that’s why I record the college kids above me.they are students with basketball feet constant hard thumps and yelling .
Hi Derrick. I just read your post and I thought your comment regarding noise recording was interesting. I am also experiencing similar issues in my apartment. Can you advise on a good recording device to record nuisance neighbours?. I have tried recording on my Huawei P30 Lite but the quality does not capture the true extent of the sound. I’d be interested to know what you used if you don’t mind sharing. Regards Phil (London)
Thanks for commenting! To record nuisance noise from neighbors, a device with high sensitivity and quality microphones is key. Smartphones like the Huawei P30 Lite often don’t capture low frequencies or varying sound levels effectively. I’d recommend using a digital voice recorder designed for sound clarity and range. These devices have built-in stereo microphones that can capture a wider range of sounds more accurately, including subtle noises and bass frequencies that a phone might miss. For more details, check out this article .
Leave a Comment Cancel Reply
Your email address will not be published. Required fields are marked *
- Random article
- Teaching guide
- Privacy & cookies
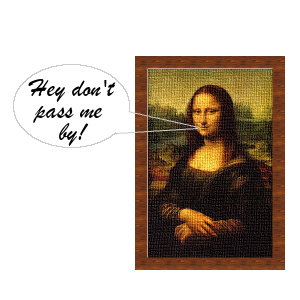
by Chris Woodford . Last updated: September 13, 2023.
Photo: Thanks to directional speakers, museum exhibits could soon be talking to you—and only you!.
What is a directional loudspeaker?
Photo: U.S. Army soldiers send a warning with a directional speaker system called the Long Range Acoustic Device® (LRAD®). Photo by Bobby L. Allen Jr. courtesy of U.S. Army.
Photo: Shining a sound spotlight. Army personnel practice riot control techniques with an LRAD® speaker mounted on the back of a truck. Photo by David Overson courtesy of US Army and DVIDS .
How directional speakers work—in simple terms
Photo: Energy ripples out in waves across the surface of water. The further the waves travel, the more their energy dissipates, because it's spread further, over a widening area.
Photo: A conventional (electromagnetic) speaker has a single, large, sound-producing cone.
How directional speakers work—a more complex explanation
Summary of how directional speakers work, what are directional speakers used for.
Photo: A Long Range Acoustic Device (LRAD®) is being used here to send a warning from the USS Blue Ridge to a small, incoming craft during an attack drill. Note how you have to swivel the LRAD® and point it in the direction you want the sound to go, just like a flashlight. Photo by Tucker M. Yates courtesy of US Navy and Wikimedia Commons .
Photo: Front and back views of two different LRAD® models. Left: You can get a sense of how big and bulky this LRAD is: it's being carried here by two men. Photo by Jordan Kirkjohnson courtesy of US Navy . U.S. Navy . --> Right: Here's the back of a lightweight LRAD 500X (Extreme) with the control panel and microphone unit just underneath. This model is 64cm (24in) square and can beam sounds up to 2km (1.2 miles) away. Photo by Amanda Dunford courtesy of US Navy .
Photo: The control panel and microphone around the back of an LRAD®1000Xi speaker, which can throw 153dB of sound at a distance of up to 3km (~1.9 miles). Photo by Riley McDowell courtesy of US Navy and DVIDS .
Who invented directional speakers?
If you liked this article..., don't want to read our articles try listening instead, find out more, on this website.
- Loudspeakers
On other sites
News articles.
Text copyright © Chris Woodford 2009, 2023. All rights reserved. Full copyright notice and terms of use .
Rate this page
Tell your friends, cite this page, more to explore on our website....
- Get the book
- Send feedback
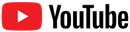
- TPC and eLearning
- What's NEW at TPC?
- Read Watch Interact
- Practice Review Test
- Teacher-Tools
- Request a Demo
- Get A Quote
- Subscription Selection
- Seat Calculator
- Ad Free Account
- Edit Profile Settings
- Metric Conversions Questions
- Metric System Questions
- Metric Estimation Questions
- Significant Digits Questions
- Proportional Reasoning
- Acceleration
- Distance-Displacement
- Dots and Graphs
- Graph That Motion
- Match That Graph
- Name That Motion
- Motion Diagrams
- Pos'n Time Graphs Numerical
- Pos'n Time Graphs Conceptual
- Up And Down - Questions
- Balanced vs. Unbalanced Forces
- Change of State
- Force and Motion
- Mass and Weight
- Match That Free-Body Diagram
- Net Force (and Acceleration) Ranking Tasks
- Newton's Second Law
- Normal Force Card Sort
- Recognizing Forces
- Air Resistance and Skydiving
- Solve It! with Newton's Second Law
- Which One Doesn't Belong?
- Component Addition Questions
- Head-to-Tail Vector Addition
- Projectile Mathematics
- Trajectory - Angle Launched Projectiles
- Trajectory - Horizontally Launched Projectiles
- Vector Addition
- Vector Direction
- Which One Doesn't Belong? Projectile Motion
- Forces in 2-Dimensions
- Being Impulsive About Momentum
- Explosions - Law Breakers
- Hit and Stick Collisions - Law Breakers
- Case Studies: Impulse and Force
- Impulse-Momentum Change Table
- Keeping Track of Momentum - Hit and Stick
- Keeping Track of Momentum - Hit and Bounce
- What's Up (and Down) with KE and PE?
- Energy Conservation Questions
- Energy Dissipation Questions
- Energy Ranking Tasks
- LOL Charts (a.k.a., Energy Bar Charts)
- Match That Bar Chart
- Words and Charts Questions
- Name That Energy
- Stepping Up with PE and KE Questions
- Case Studies - Circular Motion
- Circular Logic
- Forces and Free-Body Diagrams in Circular Motion
- Gravitational Field Strength
- Universal Gravitation
- Angular Position and Displacement
- Linear and Angular Velocity
- Angular Acceleration
- Rotational Inertia
- Balanced vs. Unbalanced Torques
- Getting a Handle on Torque
- Torque-ing About Rotation
- Properties of Matter
- Fluid Pressure
- Buoyant Force
- Sinking, Floating, and Hanging
- Pascal's Principle
- Flow Velocity
- Bernoulli's Principle
- Balloon Interactions
- Charge and Charging
- Charge Interactions
- Charging by Induction
- Conductors and Insulators
- Coulombs Law
- Electric Field
- Electric Field Intensity
- Polarization
- Case Studies: Electric Power
- Know Your Potential
- Light Bulb Anatomy
- I = ∆V/R Equations as a Guide to Thinking
- Parallel Circuits - ∆V = I•R Calculations
- Resistance Ranking Tasks
- Series Circuits - ∆V = I•R Calculations
- Series vs. Parallel Circuits
- Equivalent Resistance
- Period and Frequency of a Pendulum
- Pendulum Motion: Velocity and Force
- Energy of a Pendulum
- Period and Frequency of a Mass on a Spring
- Horizontal Springs: Velocity and Force
- Vertical Springs: Velocity and Force
- Energy of a Mass on a Spring
- Decibel Scale
- Frequency and Period
- Closed-End Air Columns
- Name That Harmonic: Strings
- Rocking the Boat
- Wave Basics
- Matching Pairs: Wave Characteristics
- Wave Interference
- Waves - Case Studies
- Color Addition and Subtraction
- Color Filters
- If This, Then That: Color Subtraction
- Light Intensity
- Color Pigments
- Converging Lenses
- Curved Mirror Images
- Law of Reflection
- Refraction and Lenses
- Total Internal Reflection
- Who Can See Who?
- Lab Equipment
- Lab Procedures
- Formulas and Atom Counting
- Atomic Models
- Bond Polarity
- Entropy Questions
- Cell Voltage Questions
- Heat of Formation Questions
- Reduction Potential Questions
- Oxidation States Questions
- Measuring the Quantity of Heat
- Hess's Law
- Oxidation-Reduction Questions
- Galvanic Cells Questions
- Thermal Stoichiometry
- Molecular Polarity
- Quantum Mechanics
- Balancing Chemical Equations
- Bronsted-Lowry Model of Acids and Bases
- Classification of Matter
- Collision Model of Reaction Rates
- Density Ranking Tasks
- Dissociation Reactions
- Complete Electron Configurations
- Elemental Measures
- Enthalpy Change Questions
- Equilibrium Concept
- Equilibrium Constant Expression
- Equilibrium Calculations - Questions
- Equilibrium ICE Table
- Intermolecular Forces Questions
- Ionic Bonding
- Lewis Electron Dot Structures
- Limiting Reactants
- Line Spectra Questions
- Mass Stoichiometry
- Measurement and Numbers
- Metals, Nonmetals, and Metalloids
- Metric Estimations
- Metric System
- Molarity Ranking Tasks
- Mole Conversions
- Name That Element
- Names to Formulas
- Names to Formulas 2
- Nuclear Decay
- Particles, Words, and Formulas
- Periodic Trends
- Precipitation Reactions and Net Ionic Equations
- Pressure Concepts
- Pressure-Temperature Gas Law
- Pressure-Volume Gas Law
- Chemical Reaction Types
- Significant Digits and Measurement
- States Of Matter Exercise
- Stoichiometry Law Breakers
- Stoichiometry - Math Relationships
- Subatomic Particles
- Spontaneity and Driving Forces
- Gibbs Free Energy
- Volume-Temperature Gas Law
- Acid-Base Properties
- Energy and Chemical Reactions
- Chemical and Physical Properties
- Valence Shell Electron Pair Repulsion Theory
- Writing Balanced Chemical Equations
- Mission CG1
- Mission CG10
- Mission CG2
- Mission CG3
- Mission CG4
- Mission CG5
- Mission CG6
- Mission CG7
- Mission CG8
- Mission CG9
- Mission EC1
- Mission EC10
- Mission EC11
- Mission EC12
- Mission EC2
- Mission EC3
- Mission EC4
- Mission EC5
- Mission EC6
- Mission EC7
- Mission EC8
- Mission EC9
- Mission RL1
- Mission RL2
- Mission RL3
- Mission RL4
- Mission RL5
- Mission RL6
- Mission KG7
- Mission RL8
- Mission KG9
- Mission RL10
- Mission RL11
- Mission RM1
- Mission RM2
- Mission RM3
- Mission RM4
- Mission RM5
- Mission RM6
- Mission RM8
- Mission RM10
- Mission LC1
- Mission RM11
- Mission LC2
- Mission LC3
- Mission LC4
- Mission LC5
- Mission LC6
- Mission LC8
- Mission SM1
- Mission SM2
- Mission SM3
- Mission SM4
- Mission SM5
- Mission SM6
- Mission SM8
- Mission SM10
- Mission KG10
- Mission SM11
- Mission KG2
- Mission KG3
- Mission KG4
- Mission KG5
- Mission KG6
- Mission KG8
- Mission KG11
- Mission F2D1
- Mission F2D2
- Mission F2D3
- Mission F2D4
- Mission F2D5
- Mission F2D6
- Mission KC1
- Mission KC2
- Mission KC3
- Mission KC4
- Mission KC5
- Mission KC6
- Mission KC7
- Mission KC8
- Mission AAA
- Mission SM9
- Mission LC7
- Mission LC9
- Mission NL1
- Mission NL2
- Mission NL3
- Mission NL4
- Mission NL5
- Mission NL6
- Mission NL7
- Mission NL8
- Mission NL9
- Mission NL10
- Mission NL11
- Mission NL12
- Mission MC1
- Mission MC10
- Mission MC2
- Mission MC3
- Mission MC4
- Mission MC5
- Mission MC6
- Mission MC7
- Mission MC8
- Mission MC9
- Mission RM7
- Mission RM9
- Mission RL7
- Mission RL9
- Mission SM7
- Mission SE1
- Mission SE10
- Mission SE11
- Mission SE12
- Mission SE2
- Mission SE3
- Mission SE4
- Mission SE5
- Mission SE6
- Mission SE7
- Mission SE8
- Mission SE9
- Mission VP1
- Mission VP10
- Mission VP2
- Mission VP3
- Mission VP4
- Mission VP5
- Mission VP6
- Mission VP7
- Mission VP8
- Mission VP9
- Mission WM1
- Mission WM2
- Mission WM3
- Mission WM4
- Mission WM5
- Mission WM6
- Mission WM7
- Mission WM8
- Mission WE1
- Mission WE10
- Mission WE2
- Mission WE3
- Mission WE4
- Mission WE5
- Mission WE6
- Mission WE7
- Mission WE8
- Mission WE9
- Vector Walk Interactive
- Name That Motion Interactive
- Kinematic Graphing 1 Concept Checker
- Kinematic Graphing 2 Concept Checker
- Graph That Motion Interactive
- Two Stage Rocket Interactive
- Rocket Sled Concept Checker
- Force Concept Checker
- Free-Body Diagrams Concept Checker
- Free-Body Diagrams The Sequel Concept Checker
- Skydiving Concept Checker
- Elevator Ride Concept Checker
- Vector Addition Concept Checker
- Vector Walk in Two Dimensions Interactive
- Name That Vector Interactive
- River Boat Simulator Concept Checker
- Projectile Simulator 2 Concept Checker
- Projectile Simulator 3 Concept Checker
- Hit the Target Interactive
- Turd the Target 1 Interactive
- Turd the Target 2 Interactive
- Balance It Interactive
- Go For The Gold Interactive
- Egg Drop Concept Checker
- Fish Catch Concept Checker
- Exploding Carts Concept Checker
- Collision Carts - Inelastic Collisions Concept Checker
- Its All Uphill Concept Checker
- Stopping Distance Concept Checker
- Chart That Motion Interactive
- Roller Coaster Model Concept Checker
- Uniform Circular Motion Concept Checker
- Horizontal Circle Simulation Concept Checker
- Vertical Circle Simulation Concept Checker
- Race Track Concept Checker
- Gravitational Fields Concept Checker
- Orbital Motion Concept Checker
- Angular Acceleration Concept Checker
- Balance Beam Concept Checker
- Torque Balancer Concept Checker
- Aluminum Can Polarization Concept Checker
- Charging Concept Checker
- Name That Charge Simulation
- Coulomb's Law Concept Checker
- Electric Field Lines Concept Checker
- Put the Charge in the Goal Concept Checker
- Circuit Builder Concept Checker (Series Circuits)
- Circuit Builder Concept Checker (Parallel Circuits)
- Circuit Builder Concept Checker (∆V-I-R)
- Circuit Builder Concept Checker (Voltage Drop)
- Equivalent Resistance Interactive
- Pendulum Motion Simulation Concept Checker
- Mass on a Spring Simulation Concept Checker
- Particle Wave Simulation Concept Checker
- Boundary Behavior Simulation Concept Checker
- Slinky Wave Simulator Concept Checker
- Simple Wave Simulator Concept Checker
- Wave Addition Simulation Concept Checker
- Standing Wave Maker Simulation Concept Checker
- Color Addition Concept Checker
- Painting With CMY Concept Checker
- Stage Lighting Concept Checker
- Filtering Away Concept Checker
- InterferencePatterns Concept Checker
- Young's Experiment Interactive
- Plane Mirror Images Interactive
- Who Can See Who Concept Checker
- Optics Bench (Mirrors) Concept Checker
- Name That Image (Mirrors) Interactive
- Refraction Concept Checker
- Total Internal Reflection Concept Checker
- Optics Bench (Lenses) Concept Checker
- Kinematics Preview
- Velocity Time Graphs Preview
- Moving Cart on an Inclined Plane Preview
- Stopping Distance Preview
- Cart, Bricks, and Bands Preview
- Fan Cart Study Preview
- Friction Preview
- Coffee Filter Lab Preview
- Friction, Speed, and Stopping Distance Preview
- Up and Down Preview
- Projectile Range Preview
- Ballistics Preview
- Juggling Preview
- Marshmallow Launcher Preview
- Air Bag Safety Preview
- Colliding Carts Preview
- Collisions Preview
- Engineering Safer Helmets Preview
- Push the Plow Preview
- Its All Uphill Preview
- Energy on an Incline Preview
- Modeling Roller Coasters Preview
- Hot Wheels Stopping Distance Preview
- Ball Bat Collision Preview
- Energy in Fields Preview
- Weightlessness Training Preview
- Roller Coaster Loops Preview
- Universal Gravitation Preview
- Keplers Laws Preview
- Kepler's Third Law Preview
- Charge Interactions Preview
- Sticky Tape Experiments Preview
- Wire Gauge Preview
- Voltage, Current, and Resistance Preview
- Light Bulb Resistance Preview
- Series and Parallel Circuits Preview
- Thermal Equilibrium Preview
- Linear Expansion Preview
- Heating Curves Preview
- Electricity and Magnetism - Part 1 Preview
- Electricity and Magnetism - Part 2 Preview
- Vibrating Mass on a Spring Preview
- Period of a Pendulum Preview
- Wave Speed Preview
- Slinky-Experiments Preview
- Standing Waves in a Rope Preview
- Sound as a Pressure Wave Preview
- DeciBel Scale Preview
- DeciBels, Phons, and Sones Preview
- Sound of Music Preview
- Shedding Light on Light Bulbs Preview
- Models of Light Preview
- Electromagnetic Radiation Preview
- Electromagnetic Spectrum Preview
- EM Wave Communication Preview
- Digitized Data Preview
- Light Intensity Preview
- Concave Mirrors Preview
- Object Image Relations Preview
- Snells Law Preview
- Reflection vs. Transmission Preview
- Magnification Lab Preview
- Reactivity Preview
- Ions and the Periodic Table Preview
- Periodic Trends Preview
- Chemical Reactions Preview
- Intermolecular Forces Preview
- Melting Points and Boiling Points Preview
- Bond Energy and Reactions Preview
- Reaction Rates Preview
- Ammonia Factory Preview
- Stoichiometry Preview
- Nuclear Chemistry Preview
- Gaining Teacher Access
- Task Tracker Directions
- Conceptual Physics Course
- On-Level Physics Course
- Honors Physics Course
- Chemistry Concept Builders
- All Chemistry Resources
- Users Voice
- Tasks and Classes
- Webinars and Trainings
- Subscription
- Subscription Locator
- 1-D Kinematics
- Newton's Laws
- Vectors - Motion and Forces in Two Dimensions
- Momentum and Its Conservation
- Work and Energy
- Circular Motion and Satellite Motion
- Thermal Physics
- Static Electricity
- Electric Circuits
- Vibrations and Waves
- Sound Waves and Music
- Light and Color
- Reflection and Mirrors
- Measurement and Calculations
- Elements, Atoms, and Ions
- About the Physics Interactives
- Task Tracker
- Usage Policy
- Newtons Laws
- Vectors and Projectiles
- Forces in 2D
- Momentum and Collisions
- Circular and Satellite Motion
- Balance and Rotation
- Electromagnetism
- Waves and Sound
- Atomic Physics
- Forces in Two Dimensions
- Work, Energy, and Power
- Circular Motion and Gravitation
- Sound Waves
- 1-Dimensional Kinematics
- Circular, Satellite, and Rotational Motion
- Einstein's Theory of Special Relativity
- Waves, Sound and Light
- QuickTime Movies
- About the Concept Builders
- Pricing For Schools
- Directions for Version 2
- Measurement and Units
- Relationships and Graphs
- Rotation and Balance
- Vibrational Motion
- Reflection and Refraction
- Teacher Accounts
- Kinematic Concepts
- Kinematic Graphing
- Wave Motion
- Sound and Music
- About CalcPad
- 1D Kinematics
- Vectors and Forces in 2D
- Simple Harmonic Motion
- Rotational Kinematics
- Rotation and Torque
- Rotational Dynamics
- Electric Fields, Potential, and Capacitance
- Transient RC Circuits
- Light Waves
- Units and Measurement
- Stoichiometry
- Molarity and Solutions
- Thermal Chemistry
- Acids and Bases
- Kinetics and Equilibrium
- Solution Equilibria
- Oxidation-Reduction
- Nuclear Chemistry
- Newton's Laws of Motion
- Work and Energy Packet
- Static Electricity Review
- NGSS Alignments
- 1D-Kinematics
- Projectiles
- Circular Motion
- Magnetism and Electromagnetism
- Graphing Practice
- About the ACT
- ACT Preparation
- For Teachers
- Other Resources
- Solutions Guide
- Solutions Guide Digital Download
- Motion in One Dimension
- Work, Energy and Power
- Chemistry of Matter
- Measurement and the Metric System
- Names and Formulas
- Algebra Based On-Level Physics
- Honors Physics
- Conceptual Physics
- Other Tools
- Frequently Asked Questions
- Purchasing the Download
- Purchasing the Digital Download
- About the NGSS Corner
- NGSS Search
- Force and Motion DCIs - High School
- Energy DCIs - High School
- Wave Applications DCIs - High School
- Force and Motion PEs - High School
- Energy PEs - High School
- Wave Applications PEs - High School
- Crosscutting Concepts
- The Practices
- Physics Topics
- NGSS Corner: Activity List
- NGSS Corner: Infographics
- About the Toolkits
- Position-Velocity-Acceleration
- Position-Time Graphs
- Velocity-Time Graphs
- Newton's First Law
- Newton's Second Law
- Newton's Third Law
- Terminal Velocity
- Projectile Motion
- Forces in 2 Dimensions
- Impulse and Momentum Change
- Momentum Conservation
- Work-Energy Fundamentals
- Work-Energy Relationship
- Roller Coaster Physics
- Satellite Motion
- Electric Fields
- Circuit Concepts
- Series Circuits
- Parallel Circuits
- Describing-Waves
- Wave Behavior Toolkit
- Standing Wave Patterns
- Resonating Air Columns
- Wave Model of Light
- Plane Mirrors
- Curved Mirrors
- Teacher Guide
- Using Lab Notebooks
- Current Electricity
- Light Waves and Color
- Reflection and Ray Model of Light
- Refraction and Ray Model of Light
- Teacher Resources
- Subscriptions

- Newton's Laws
- Einstein's Theory of Special Relativity
- About Concept Checkers
- School Pricing
- Newton's Laws of Motion
- Newton's First Law
- Newton's Third Law
- Sound is a Pressure Wave
- Sound is a Mechanical Wave
- Sound is a Longitudinal Wave
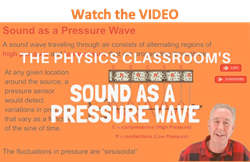
Compressions and Rarefactions
A vibrating tuning fork is capable of creating such a longitudinal wave. As the tines of the fork vibrate back and forth, they push on neighboring air particles. The forward motion of a tine pushes air molecules horizontally to the right and the backward retraction of the tine creates a low-pressure area allowing the air particles to move back to the left.
Because of the longitudinal motion of the air particles, there are regions in the air where the air particles are compressed together and other regions where the air particles are spread apart. These regions are known as compressions and rarefactions respectively. The compressions are regions of high air pressure while the rarefactions are regions of low air pressure. The diagram below depicts a sound wave created by a tuning fork and propagated through the air in an open tube. The compressions and rarefactions are labeled.
The wavelength of a wave is merely the distance that a disturbance travels along the medium in one complete wave cycle. Since a wave repeats its pattern once every wave cycle, the wavelength is sometimes referred to as the length of the repeating patterns - the length of one complete wave. For a transverse wave, this length is commonly measured from one wave crest to the next adjacent wave crest or from one wave trough to the next adjacent wave trough. Since a longitudinal wave does not contain crests and troughs, its wavelength must be measured differently. A longitudinal wave consists of a repeating pattern of compressions and rarefactions. Thus, the wavelength is commonly measured as the distance from one compression to the next adjacent compression or the distance from one rarefaction to the next adjacent rarefaction.
What is a Pressure Wave?
Since a sound wave consists of a repeating pattern of high-pressure and low-pressure regions moving through a medium, it is sometimes referred to as a pressure wave . If a detector, whether it is the human ear or a man-made instrument, were used to detect a sound wave, it would detect fluctuations in pressure as the sound wave impinges upon the detecting device. At one instant in time, the detector would detect a high pressure; this would correspond to the arrival of a compression at the detector site. At the next instant in time, the detector might detect normal pressure. And then finally a low pressure would be detected, corresponding to the arrival of a rarefaction at the detector site. The fluctuations in pressure as detected by the detector occur at periodic and regular time intervals. In fact, a plot of pressure versus time would appear as a sine curve. The peak points of the sine curve correspond to compressions; the low points correspond to rarefactions; and the "zero points" correspond to the pressure that the air would have if there were no disturbance moving through it. The diagram below depicts the correspondence between the longitudinal nature of a sound wave in air and the pressure-time fluctuations that it creates at a fixed detector location.
The above diagram can be somewhat misleading if you are not careful. The representation of sound by a sine wave is merely an attempt to illustrate the sinusoidal nature of the pressure-time fluctuations. Do not conclude that sound is a transverse wave that has crests and troughs. Sound waves traveling through air are indeed longitudinal waves with compressions and rarefactions. As sound passes through air (or any fluid medium), the particles of air do not vibrate in a transverse manner. Do not be misled - sound waves traveling through air are longitudinal waves.
We Would Like to Suggest ...
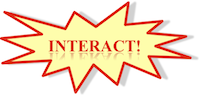
Check Your Understanding
1. A sound wave is a pressure wave; regions of high (compressions) and low pressure (rarefactions) are established as the result of the vibrations of the sound source. These compressions and rarefactions result because sound
a. is more dense than air and thus has more inertia, causing the bunching up of sound. b. waves have a speed that is dependent only upon the properties of the medium. c. is like all waves; it is able to bend into the regions of space behind obstacles. d. is able to reflect off fixed ends and interfere with incident waves e. vibrates longitudinally; the longitudinal movement of air produces pressure fluctuations.
Since the particles of the medium vibrate in a longitudinal fashion, compressions and rarefactions are created. Study the tuning fork animation provided on the Tutorial page.
- Pitch and Frequency
Want to create or adapt books like this? Learn more about how Pressbooks supports open publishing practices.
Traveling waves
17 How sound moves
Speed of sound.
There’s a delay between when a sound is created and when it is heard. In everyday life, the delay is usually too short to notice. However, the delay can be noticeable if the distance between source and detector is large enough. You see lightning before you hear the thunder. If you’ve sat in the outfield seats in a baseball stadium, you’ve experienced the delay between seeing the player hit the ball and the sound of the “whack.” Life experiences tell us that sound travels fast, but not nearly as fast as light does. Careful experiments confirm this idea.
The speed of sound in air is roughly 340 m/s. The actual value depends somewhat on the temperature and humidity. In everyday terms, sound travels about the length of three and a half foot ball fields every second- about 50% faster than a Boeing 747 (roughly 250 m/s). This may seem fast, but it’s tiny compared to light, which travels roughly a million times faster than sound (roughly 300,000,000 m/s).
Sound requires some material in which to propagate (i.e. travel). This material sound travels through is called the medium . You can show that sound requires a medium by putting a cell phone inside a glass jar connected to a vacuum pump. As the air is removed from the jar, the cell phone’s ringer gets quieter and quieter. A youTube video (2:05 min) produced by the UNSW PhysClips project shows the demo with a drumming toy monkey [1] instead of a cell phone.
What affects the speed of sound?
Sound travels at different speeds though different materials. The physical properties of the medium are the only factors that affect the speed of sound- nothing else matters.
The speed of sound in a material is determined mainly by two properties- the stiffness of the material and the density of the material. Sound travels fastest through materials that are stiff and light. In general, sound travels fastest through solids, slower through liquids and slowest through gasses. (See the table on this page). This may seem backwards- after all, metals are quite dense. However, the high density of metals is more than offset by far greater stiffness (compared to liquids and solids).
The speed of sound in air depends mainly on temperature. The speed of sound is 331 m/s in dry air at 0 o Celsius and increases slightly with temperature- about 0.6 m/s for every 1 o Celsius for temperatures commonly found on Earth. Though speed of sound in air also depends on humidity, the effect is tiny- sound travels only about 1 m/s faster in air with 100% humidity air at 20 o C than it does in completely dry air at the same temperature.
Nothing else matters
The properties of the medium are the only factors that affect the speed of sound- nothing else matters.
Frequency of the sound does not matter- high frequency sounds travel at the same speed as low frequency sounds. If you’ve ever listened to music, you’ve witnessed this- the low notes and the high notes that were made simultaneously reach you simultaneously, even if you are far from the stage. If you’ve heard someone shout from across a field, you’ve noticed that the entire shout sound (which contains many different frequencies at once) reaches you at the same time. If different frequencies traveled at different rates, some frequencies would arrive before others.
The amplitude of the sound does not matter- loud sounds and quiet ones travel at the same speed. Whisper or yell- it doesn’t matter. The sound still takes the same amount of time to reach the listener. You’ve probably heard that you can figure out how far away the lightning by counting the seconds between when you see lightning and hear thunder. If the speed of sound depended on loudness, this rule of thumb would have to account for loudness- yet there is nothing in the rule about loud vs. quiet thunder. The rule of thumb works the same for all thunder- regardless of loudness . That’s because the speed of sound doesn’t depend on amplitude.
Stop to thinks
- Which takes longer to cross a football field: the sound of a high pitched chirp emitted by a fruit bat or the (relatively) low pitched sound emitted by a trumpet?
- Which sound takes longer to travel 100 meters: the sound of a snapping twig in the forest or the sound of a gunshot?
- Which takes longer to travel the distance of a football field: the low pitched sound of a whale or the somewhat higher pitched sound of a human being?
Constant speed
Sound travels at a constant speed. Sound does not speed up or slow down as it travels (unless the properties of the material the sound is going through changes). I know what you’re thinking- how is that possible? Sounds die out as they travel, right? True. That means sounds must slow down and come to a stop, right? Wrong. As sound travels, its amplitude decreases- but that’s not the same thing as slowing down. Sound (in air) covers roughly 340 meters each and every second, even as its amplitude shrinks. Eventually, the amplitude gets small enough that the sound is undetectable. A sound’s amplitude shrinks as it travels, but its speed remains constant.
The basic equation for constant speed motion (shown below) applies to sound.
[latex]d=vt[/latex]
In this equation, [latex]d[/latex] represents the distance traveled by the sound, [latex]t[/latex] represents the amount of time it took to go that distance and [latex]v[/latex] represents the speed.
Rule of thumb for lightning example
Example: thunder and lightning.
The rule of thumb for figuring out how far away a lightning strike is from you is this:
Count the number of seconds between when you see the lightning and hear the thunder. Divide the number of seconds by five to find out how many miles away the lightning hit.
According to this rule, what is the speed of sound in air? How does the speed of sound implied by this rule compare to 340 m/s?
Identify important physics concept : This question is about speed of sound.
List known and unknown quantities (with letter names and units):
At first glance, it doesn’t look like there’s enough information to solve the problem. We were asked to find speed, but not given either a time or a distance. However, the problem does allow us to figure out a distance if we know the time- “Divide the number of seconds by five to find out how many miles away the lightning hit.” So, let’s make up a time and see what happens; if the time is 10 seconds, the rule of thumb says that the distance should be 2 miles.
[latex]v= \: ?[/latex]
[latex]d=2 \: miles[/latex]
[latex]t=10 \: seconds[/latex]
You might ask “Is making stuff up OK here?” The answer is YES! If the rule of thumb is right, it should work no matter what time we choose. (To check if the rule is OK, we should probably test it with more than just one distance-time combination, but we’ll assume the rule is OK for now).
Do the algebra: The equation is already solved for speed. Move on to the next step.
Do unit conversions (if needed) then plug in numbers: If you just plug in the numbers, the speed comes out in miles per second:
[latex]v = \frac{2 \: miles} {10 \: seconds}=0.2 \: \frac{miles} {second}[/latex]
We are asked to compare this speed to 340 m/s, so a unit conversion is in order; since there are 1609 meters in a mile:
[latex]v =0.2 \: \frac{miles} {second}*\frac{1609 \: meters} {1 \:mile}=320 \frac{m}{s}[/latex]
Reflect on the answer:
- The answer for speed from the rule of thumb is less than 10% off the actual value of roughly 340 m/s- surprisingly close!
- At the beginning, we assumed a time of 10 seconds. Does the result hold up for other choices? A quick check shows that it does! For instance, if we use a time of 5 seconds, the rule of thumb gives a distance of 1 mile, and the math still gives a speed of 0.2 miles/second. The speed will be the same no matter what time we pick. The reason is this: The more time it takes the thunder to arrive, the farther away the lightning strike is, but the speed remains the same. In the equation for speed, both time and distance change by the same factor and the overall fraction remains unchanged.
Stop to think answers
- Both sounds take the same amount of time. (High and low pitched sounds travel at the same speed).
- Both sounds take the same amount of time. (Quiet sounds and loud sounds travel at the same speed).
- The sound of the whale travels the distance in less time- assuming sound from the whale travels in water and sound from the human travels in air. Sound travels faster in water than in air. (The info about frequency was given just to throw you off- frequency doesn’t matter).
- Wolfe, J. (2014, February 20). Properties of Sound. Retrieved from https://www.youtube.com/watch?v=P8-govgAffY ↵
Understanding Sound Copyright © by dsa2gamba and abbottds is licensed under a Creative Commons Attribution 4.0 International License , except where otherwise noted.
Share This Book
Researchers make sound waves travel in one direction only, with implications for electromagnetic wave technology
R esearchers at ETH Zurich have managed to make sound waves travel only in one direction. In the future, this method could also be used in technical applications with electromagnetic waves.
Water, light and sound waves usually propagate in the same way forward as in a backward direction. As a consequence, when we are speaking to someone standing some distance away from us, that person can hear us as well as we can hear them. This is useful when having a conversation, but in some technical applications one would prefer the waves to be able to travel only in one direction—for instance, in order to avoid unwanted reflections of light or microwaves.
Ten years ago, researchers succeeded in suppressing sound wave propagation in the backward direction; however, this also attenuated the waves traveling forwards.
A team of researchers at ETH Zurich led by Nicolas Noiray, professor for Combustion, Acoustics and Flow Physics, in collaboration with Romain Fleury at EPFL, has now developed a method for preventing sound waves from traveling backward without deteriorating their propagation in the forward direction.
In the future, this method, which has recently been published in Nature Communications , could also be applied to electromagnetic waves.
The basis of this one-way street for sound waves are self-oscillations, in which a dynamical system periodically repeats its behavior. "I've actually spent a good part of my career preventing such phenomena," says Noiray.
Among other things, he studies how self-sustaining thermo-acoustic oscillations can arise from the interplay between sound waves and flames in the combustion chamber of an aircraft engine, which can lead to dangerous vibrations. In the worst case, these vibrations can destroy the engine.
Harmless and useful self-oscillations
Noiray had the idea to use harmless self-sustaining aero-acoustic oscillations in order to allow sound waves to pass only in one direction and without any losses through a so-called circulator. In his scheme, the unavoidable attenuation of the sound waves is compensated by the self-oscillations in the circulator synchronizing with the incoming waves, which allows them to gain energy from those oscillations.
The circulator itself was supposed to consist of a disk-shaped cavity through which swirling air is blown from one side through an opening in its center. For a specific combination of blowing speed and intensity of the swirl, a whistling sound is thus created in the cavity.
"In contrast to ordinary whistles, in which sound is created by a standing wave in the cavity, in this new whistle it results from a spinning wave," explains Tiemo Pedergnana, a former doctoral student in Noiray's group and lead author of the study.
From the idea to the experiment, it took a while. First, Noiray and his co-workers investigated the fluid mechanics of the spinning wave whistle, and then added three acoustic waveguides to it, which are arranged in a triangular shape along the edge of the circulator.
Sound waves that are fed in through the first waveguide can leave the circulator through the second waveguide. However, a wave entering through the second waveguide cannot exit "backwards" through the first waveguide, but can do so through the third waveguide.
Sound waves as a toy model
Over several years, the ETH researchers developed and theoretically modeled the various parts of the circulator; now, finally, they could experimentally demonstrate that their loss-compensation approach works. They sent a sound wave with a frequency of around 800 Hertz (roughly the high g of a soprano) through the first waveguide and measured how well it was transmitted to the second and third waveguides.
As expected, the sound wave didn't make it to the third waveguide. From the second waveguide (in the "forward" direction), however, a sound wave emerged that was even stronger than the one originally sent in.
"This concept of loss-compensated non-reciprocal wave propagation is, in our view, an important result that can also be transferred to other systems," says Noiray. He sees his sound wave circulator mainly as a powerful toy model for the general approach of wave manipulation using synchronized self-oscillations that can, for instance, be applied to metamaterials for electromagnetic waves.
In this way, microwaves in radar systems could be guided better, and so-called topological circuits could be realized, with which signals can be routed in future communications systems.
More information: Tiemo Pedergnana et al, Loss-compensated non-reciprocal scattering based on synchronization, Nature Communications (2024). DOI: 10.1038/s41467-024-51373-y
Provided by ETH Zurich
14.1 Speed of Sound, Frequency, and Wavelength
Section learning objectives.
By the end of this section, you will be able to do the following:
- Relate the characteristics of waves to properties of sound waves
- Describe the speed of sound and how it changes in various media
- Relate the speed of sound to frequency and wavelength of a sound wave
Teacher Support
The learning objectives in this section will help your students master the following standards:
- (A) examine and describe oscillatory motion and wave propagation in various types of media;
- (B) investigate and analyze characteristics of waves, including velocity, frequency, amplitude, and wavelength, and calculate using the relationship between wave speed, frequency, and wavelength;
- (C) compare characteristics and behaviors of transverse waves, including electromagnetic waves and the electromagnetic spectrum, and characteristics and behaviors of longitudinal waves, including sound waves;
- (F) describe the role of wave characteristics and behaviors in medical and industrial applications.
In addition, the High School Physics Laboratory Manual addresses content in this section in the lab titled: Waves, as well as the following standards:
- (B) investigate and analyze characteristics of waves, including velocity, frequency, amplitude, and wavelength, and calculate using the relationship between wave speed, frequency, and wavelength.
Section Key Terms
[BL] [OL] Review waves and types of waves—mechanical and non-mechanical, transverse and longitudinal, pulse and periodic. Review properties of waves—amplitude, period, frequency, velocity and their inter-relations.
Properties of Sound Waves
Sound is a wave. More specifically, sound is defined to be a disturbance of matter that is transmitted from its source outward. A disturbance is anything that is moved from its state of equilibrium. Some sound waves can be characterized as periodic waves, which means that the atoms that make up the matter experience simple harmonic motion .
A vibrating string produces a sound wave as illustrated in Figure 14.2 , Figure 14.3 , and Figure 14.4 . As the string oscillates back and forth, part of the string’s energy goes into compressing and expanding the surrounding air. This creates slightly higher and lower pressures. The higher pressure... regions are compressions, and the low pressure regions are rarefactions . The pressure disturbance moves through the air as longitudinal waves with the same frequency as the string. Some of the energy is lost in the form of thermal energy transferred to the air. You may recall from the chapter on waves that areas of compression and rarefaction in longitudinal waves (such as sound) are analogous to crests and troughs in transverse waves .
The amplitude of a sound wave decreases with distance from its source, because the energy of the wave is spread over a larger and larger area. But some of the energy is also absorbed by objects, such as the eardrum in Figure 14.5 , and some of the energy is converted to thermal energy in the air. Figure 14.4 shows a graph of gauge pressure versus distance from the vibrating string. From this figure, you can see that the compression of a longitudinal wave is analogous to the peak of a transverse wave, and the rarefaction of a longitudinal wave is analogous to the trough of a transverse wave. Just as a transverse wave alternates between peaks and troughs, a longitudinal wave alternates between compression and rarefaction.
The Speed of Sound
[BL] Review the fact that sound is a mechanical wave and requires a medium through which it is transmitted.
[OL] [AL] Ask students if they know the speed of sound and if not, ask them to take a guess. Ask them why the sound of thunder is heard much after the lightning is seen during storms. This phenomenon is also observed during a display of fireworks. Through this discussion, develop the concept that the speed of sound is finite and measurable and is much slower than that of light.
The speed of sound varies greatly depending upon the medium it is traveling through. The speed of sound in a medium is determined by a combination of the medium’s rigidity (or compressibility in gases) and its density. The more rigid (or less compressible) the medium, the faster the speed of sound. The greater the density of a medium, the slower the speed of sound. The speed of sound in air is low, because air is compressible. Because liquids and solids are relatively rigid and very difficult to compress, the speed of sound in such media is generally greater than in gases. Table 14.1 shows the speed of sound in various media. Since temperature affects density, the speed of sound varies with the temperature of the medium through which it’s traveling to some extent, especially for gases.
Misconception Alert
Students might be confused between rigidity and density and how they affect the speed of sound. The speed of sound is slower in denser media. Solids are denser than gases. However, they are also very rigid, and hence sound travels faster in solids. Stress on the fact that the speed of sound always depends on a combination of these two properties of any medium.
[BL] Note that in the table, the speed of sound in very rigid materials such as glass, aluminum, and steel ... is quite high, whereas the speed in rubber, which is considerably less rigid, is quite low.
The Relationship Between the Speed of Sound and the Frequency and Wavelength of a Sound Wave
Sound, like all waves, travels at certain speeds through different media and has the properties of frequency and wavelength . Sound travels much slower than light—you can observe this while watching a fireworks display (see Figure 14.6 ), since the flash of an explosion is seen before its sound is heard.
The relationship between the speed of sound, its frequency, and wavelength is the same as for all waves:
where v is the speed of sound (in units of m/s), f is its frequency (in units of hertz), and λ λ is its wavelength (in units of meters). Recall that wavelength is defined as the distance between adjacent identical parts of a wave. The wavelength of a sound, therefore, is the distance between adjacent identical parts of a sound wave. Just as the distance between adjacent crests in a transverse wave is one wavelength, the distance between adjacent compressions in a sound wave is also one wavelength, as shown in Figure 14.7 . The frequency of a sound wave is the same as that of the source. For example, a tuning fork vibrating at a given frequency would produce sound waves that oscillate at that same frequency. The frequency of a sound is the number of waves that pass a point per unit time.
[BL] [OL] [AL] In musical instruments, shorter strings vibrate faster and hence produce sounds at higher pitches. Fret placements on instruments such as guitars, banjos, and mandolins, are mathematically determined to give the correct interval or change in pitch. When the string is pushed against the fret wire, the string is effectively shortened, changing its pitch. Ask students to experiment with strings of different lengths and observe how the pitch changes in each case.
One of the more important properties of sound is that its speed is nearly independent of frequency. If this were not the case, and high-frequency sounds traveled faster, for example, then the farther you were from a band in a football stadium, the more the sound from the low-pitch instruments would lag behind the high-pitch ones. But the music from all instruments arrives in cadence independent of distance, and so all frequencies must travel at nearly the same speed.
Recall that v = f λ v = f λ , and in a given medium under fixed temperature and humidity, v is constant. Therefore, the relationship between f and λ λ is inverse: The higher the frequency, the shorter the wavelength of a sound wave.
Teacher Demonstration
Hold a meter stick flat on a desktop, with about 80 cm sticking out over the edge of the desk. Make the meter stick vibrate by pulling the tip down and releasing, while holding the meter stick tight to the desktop. While it is vibrating, move the stick back onto the desktop, shortening the part that is sticking out. Students will see the shortening of the vibrating part of the meter stick, and hear the pitch or number of vibrations go up—an increase in frequency.
The speed of sound can change when sound travels from one medium to another. However, the frequency usually remains the same because it is like a driven oscillation and maintains the frequency of the original source. If v changes and f remains the same, then the wavelength λ λ must change. Since v = f λ v = f λ , the higher the speed of a sound, the greater its wavelength for a given frequency.
[AL] Ask students to predict what would happen if the speeds of sound in air varied by frequency.
Virtual Physics
This simulation lets you see sound waves. Adjust the frequency or amplitude (volume) and you can see and hear how the wave changes. Move the listener around and hear what she hears. Switch to the Two Source Interference tab or the Interference by Reflection tab to experiment with interference and reflection.
Tips For Success
Make sure to have audio enabled and set to Listener rather than Speaker, or else the sound will not vary as you move the listener around.
- Because, intensity of the sound wave changes with the frequency.
- Because, the speed of the sound wave changes when the frequency is changed.
- Because, loudness of the sound wave takes time to adjust after a change in frequency.
- Because it takes time for sound to reach the listener, so the listener perceives the new frequency of sound wave after a delay.
- Yes, the speed of propagation depends only on the frequency of the wave.
- Yes, the speed of propagation depends upon the wavelength of the wave, and wavelength changes as the frequency changes.
- No, the speed of propagation depends only on the wavelength of the wave.
- No, the speed of propagation is constant in a given medium; only the wavelength changes as the frequency changes.
Voice as a Sound Wave
In this lab you will observe the effects of blowing and speaking into a piece of paper in order to compare and contrast different sound waves.
- sheet of paper
Instructions
- Suspend a sheet of paper so that the top edge of the paper is fixed and the bottom edge is free to move. You could tape the top edge of the paper to the edge of a table, for example.
- Gently blow air near the edge of the bottom of the sheet and note how the sheet moves.
- Speak softly and then louder such that the sounds hit the edge of the bottom of the paper, and note how the sheet moves.
- Interpret the results.
Grasp Check
Which sound wave property increases when you are speaking more loudly than softly?
- amplitude of the wave
- frequency of the wave
- speed of the wave
- wavelength of the wave
Worked Example
What are the wavelengths of audible sounds.
Calculate the wavelengths of sounds at the extremes of the audible range, 20 and 20,000 Hz, in conditions where sound travels at 348.7 m/s.
To find wavelength from frequency, we can use v = f λ v = f λ .
(1) Identify the knowns. The values for v and f are given.
(2) Solve the relationship between speed, frequency and wavelength for λ λ .
(3) Enter the speed and the minimum frequency to give the maximum wavelength.
(4) Enter the speed and the maximum frequency to give the minimum wavelength.
Because the product of f multiplied by λ λ equals a constant velocity in unchanging conditions, the smaller f is, the larger λ λ must be, and vice versa. Note that you can also easily rearrange the same formula to find frequency or velocity.
Practice Problems
- 5 × 10 3 m / s
- 3.2 × 10 2 m / s
- 2 × 10 − 4 m/s
- 8 × 10 2 m / s
- 2.0 × 10 7 m
- 1.5 × 10 7 m
- 1.4 × 10 2 m
- 7.4 × 10 − 3 m
Links To Physics
Echolocation.
Echolocation is the use of reflected sound waves to locate and identify objects. It is used by animals such as bats, dolphins and whales, and is also imitated by humans in SONAR—Sound Navigation and Ranging—and echolocation technology.
Bats, dolphins and whales use echolocation to navigate and find food in their environment. They locate an object (or obstacle) by emitting a sound and then sensing the reflected sound waves. Since the speed of sound in air is constant, the time it takes for the sound to travel to the object and back gives the animal a sense of the distance between itself and the object. This is called ranging . Figure 14.8 shows a bat using echolocation to sense distances.
Echolocating animals identify an object by comparing the relative intensity of the sound waves returning to each ear to figure out the angle at which the sound waves were reflected. This gives information about the direction, size and shape of the object. Since there is a slight distance in position between the two ears of an animal, the sound may return to one of the ears with a bit of a delay, which also provides information about the position of the object. For example, if a bear is directly to the right of a bat, the echo will return to the bat’s left ear later than to its right ear. If, however, the bear is directly ahead of the bat, the echo would return to both ears at the same time. For an animal without a sense of sight such as a bat, it is important to know where other animals are as well as what they are; their survival depends on it.
Principles of echolocation have been used to develop a variety of useful sensing technologies. SONAR, is used by submarines to detect objects underwater and measure water depth. Unlike animal echolocation, which relies on only one transmitter (a mouth) and two receivers (ears), manmade SONAR uses many transmitters and beams to get a more accurate reading of the environment. Radar technologies use the echo of radio waves to locate clouds and storm systems in weather forecasting, and to locate aircraft for air traffic control. Some new cars use echolocation technology to sense obstacles around the car, and warn the driver who may be about to hit something (or even to automatically parallel park). Echolocation technologies and training systems are being developed to help visually impaired people navigate their everyday environments.
- The echo would return to the left ear first.
- The echo would return to the right ear first.
Check Your Understanding
Use these questions to assess student achievement of the section’s Learning Objectives. If students are struggling with a specific objective, these questions will help identify which and direct students to the relevant content.
- Rarefaction is the high-pressure region created in a medium when a longitudinal wave passes through it.
- Rarefaction is the low-pressure region created in a medium when a longitudinal wave passes through it.
- Rarefaction is the highest point of amplitude of a sound wave.
- Rarefaction is the lowest point of amplitude of a sound wave.
What sort of motion do the particles of a medium experience when a sound wave passes through it?
- Simple harmonic motion
- Circular motion
- Random motion
- Translational motion
What does the speed of sound depend on?
- The wavelength of the wave
- The size of the medium
- The frequency of the wave
- The properties of the medium
What property of a gas would affect the speed of sound traveling through it?
- The volume of the gas
- The flammability of the gas
- The mass of the gas
- The compressibility of the gas
This book may not be used in the training of large language models or otherwise be ingested into large language models or generative AI offerings without OpenStax's permission.
Want to cite, share, or modify this book? This book uses the Creative Commons Attribution License and you must attribute Texas Education Agency (TEA). The original material is available at: https://www.texasgateway.org/book/tea-physics . Changes were made to the original material, including updates to art, structure, and other content updates.
Access for free at https://openstax.org/books/physics/pages/1-introduction
- Authors: Paul Peter Urone, Roger Hinrichs
- Publisher/website: OpenStax
- Book title: Physics
- Publication date: Mar 26, 2020
- Location: Houston, Texas
- Book URL: https://openstax.org/books/physics/pages/1-introduction
- Section URL: https://openstax.org/books/physics/pages/14-1-speed-of-sound-frequency-and-wavelength
© Jun 7, 2024 Texas Education Agency (TEA). The OpenStax name, OpenStax logo, OpenStax book covers, OpenStax CNX name, and OpenStax CNX logo are not subject to the Creative Commons license and may not be reproduced without the prior and express written consent of Rice University.

IMAGES
VIDEO
COMMENTS
directions. Sound waves travel through air at a rate of 343 m/s (768 mph) at 68 degrees F. The speed of sound changes slightly at different temperatures, and dramatically in different materials (for example, in steel alloy, it travels 6000 m/s and in fresh water at 25 degrees F, it travels at 1497 m/s).
Measuring waves. All sound waves are the same: they travel through a medium by making atoms or molecules shake back and forth. But all sound waves are different too. There are loud sounds and quiet sounds, high-pitched squeaks and low-pitched rumbles, and even two instruments playing exactly the same musical note will produce sound waves that are quite different.
The answer is that it depends on the type of wave. Sound will always travel in a single direction until something blocks its route. The actual path of the sound waves is bent when they enter a new medium. If a sound wave hits a hard surface, such as a wall, it will reflect off of that surface and travel in a different direction.
sound waves in all directions. Sound waves contain energy, and we mainly sense this energy reach us with our ears. Sound travels through space in longitudinal waves. An object waves quickly back and forth, so the air around it starts to wave too. The waves can't be seen in air, but look like this when measured: Figure 1: Longitudinal Waves
At low frequencies (below around 1000 Hz), sound waves tend to travel in all directions more or less equally and bounce off objects like a rubber ball would. As frequency increases however, the directivity of sound increases as well. So high-frequency sounds are more likely to travel in a straight line between two points than low frequencies.
How Sound Waves Travel. Sound, being a longitudinal wave, makes the air molecules move in the same direction as the wave itself. For example, when a drum is hit, the drum's surface vibrates, causing the nearby air molecules to move back and forth along the line of the wave. This series of compressions (where the air molecules are close ...
In longitudinal sound waves, such as sound waves produced by a vibrating drumhead or our vocal cords, the particles of the medium move parallel to the wave's direction of travel. This movement creates areas of compression and rarefaction in the medium — be it air, water, or a solid — which our ears interpret as sound.
When sound waves travel through a medium, the particles of the medium vibrate. Vibrations reach the ear and then the brain which senses them and we recognize sound. Read on for an explanation of how sound travels. Sound is a series of compression and rarefraction waves that can travel long distances. It is produced by the vibration of the ...
Well, while many people think that sound travels in one direction, that's actually just a misconception. People imagine the waves of music that leave their speakers as going out in a straight line or in a conical shape from the speaker. But in order to have sound travel toward a particular area, you'd have to physically direct it. The best ...
Sound waves traveling through a fluid such as air travel as longitudinal waves. Particles of the fluid (i.e., air) vibrate back and forth in the direction that the sound wave is moving. This back-and-forth longitudinal motion creates a pattern of compressions (high pressure regions) and rarefactions (low pressure regions).
One of the biggest advantages of directional loudspeakers is how far they can make sound travel. In theory, sound pumping out from a conventional speaker follows what's called the inverse-square law, so doubling the distance from the speaker reduces the intensity by much more than half. But a directional speaker sends its sound in a much more ...
A sound is actually a pressure wave created by a vibrating object. In other words, you could say that when something vibrates, it creates sound. Originating from a source, these sound waves travel outwards in all directions at the same rate. As the waves travel, they bounce off and/or are absorbed by objects that lie in their path.
Sound can be modeled as a pressure wave by considering the change in pressure from average pressure, ΔP = ΔPmaxsin(kx ∓ ωt + ϕ). Δ P = Δ P max sin (k x ∓ ω t + ϕ). 17.1. This equation is similar to the periodic wave equations seen in Waves, where ΔP Δ P is the change in pressure, ΔPmax Δ P max is the maximum change in pressure ...
Sound waves traveling through a fluid such as air travel as longitudinal waves. Particles of the fluid (i.e., air) vibrate back and forth in the direction that the sound wave is moving. This back-and-forth longitudinal motion creates a pattern of compressions (high pressure regions) and rarefactions (low pressure regions). A detector of pressure at any location in the medium would detect ...
A sound's amplitude shrinks as it travels, but its speed remains constant. The basic equation for constant speed motion (shown below) applies to sound. d = vt d = v t. In this equation, d d represents the distance traveled by the sound, t t represents the amount of time it took to go that distance and v v represents the speed.
Researchers at ETH Zurich have managed to make sound waves travel only in one direction. In the future, this method could also be used in technical applications with electromagnetic waves.
Sound propagates through air or other mediums as a longitudinal wave, in which the mechanical vibration constituting the wave occurs along the direction of propagation of the wave. A longitudinal wave can be created in a coiled spring by squeezing several of the turns together to form a compression and then releasing them, allowing the compression to travel the length of the spring.
14.1. where v is the speed of sound (in units of m/s), f is its frequency (in units of hertz), and λ is its wavelength (in units of meters). Recall that wavelength is defined as the distance between adjacent identical parts of a wave. The wavelength of a sound, therefore, is the distance between adjacent identical parts of a sound wave.
If you're seeing this message, it means we're having trouble loading external resources on our website. If you're behind a web filter, please make sure that the domains *.kastatic.org and *.kasandbox.org are unblocked.
Khan Academy