Thank you for visiting nature.com. You are using a browser version with limited support for CSS. To obtain the best experience, we recommend you use a more up to date browser (or turn off compatibility mode in Internet Explorer). In the meantime, to ensure continued support, we are displaying the site without styles and JavaScript.
- View all journals
- My Account Login
- Explore content
- About the journal
- Publish with us
- Sign up for alerts
- Open access
- Published: 15 December 2020

K 2P 2.1 (TREK-1) potassium channel activation protects against hyperoxia-induced lung injury
- Tatiana Zyrianova 1 ,
- Benjamin Lopez 1 ,
- Riccardo Olcese 2 , 3 ,
- John Belperio 4 ,
- Christopher M. Waters 5 ,
- Leanne Wong 1 ,
- Victoria Nguyen 1 ,
- Sriharsha Talapaneni 1 &
- Andreas Schwingshackl 1
Scientific Reports volume 10 , Article number: 22011 ( 2020 ) Cite this article
2684 Accesses
16 Citations
3 Altmetric
Metrics details
- Cell biology
- Mechanisms of disease
No targeted therapies exist to counteract Hyperoxia (HO)-induced Acute Lung Injury (HALI). We previously found that HO downregulates alveolar K 2P 2.1 (TREK-1) K + channels, which results in worsening lung injury. This decrease in TREK-1 levels leaves a subset of channels amendable to pharmacological intervention. Therefore, we hypothesized that TREK-1 activation protects against HALI. We treated HO-exposed mice and primary alveolar epithelial cells (AECs) with the novel TREK-1 activators ML335 and BL1249, and quantified physiological, histological, and biochemical lung injury markers. We determined the effects of these drugs on epithelial TREK-1 currents, plasma membrane potential (Em), and intracellular Ca 2+ (iCa) concentrations using fluorometric assays, and blocked voltage-gated Ca 2+ channels (Ca V ) as a downstream mechanism of cytokine secretion. Once-daily, intra-tracheal injections of HO-exposed mice with ML335 or BL1249 improved lung compliance, histological lung injury scores, broncho-alveolar lavage protein levels and cell counts, and IL-6 and IP-10 concentrations. TREK-1 activation also decreased IL-6, IP-10, and CCL-2 secretion from primary AECs. Mechanistically, ML335 and BL1249 induced TREK-1 currents in AECs, counteracted HO-induced cell depolarization, and lowered iCa 2+ concentrations. In addition, CCL-2 secretion was decreased after L-type Ca V inhibition. Therefore, Em stabilization with TREK-1 activators may represent a novel approach to counteract HALI.
Similar content being viewed by others
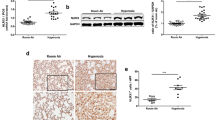
NLRX1 knockdown attenuates pro-apoptotic signaling and cell death in pulmonary hyperoxic acute injury
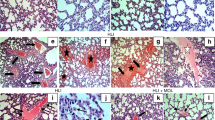
Molsidomine decreases hyperoxia-induced lung injury in neonatal rats
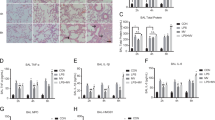
JAK2/STAT1-mediated HMGB1 translocation increases inflammation and cell death in a ventilator-induced lung injury model
Introduction.
Oxygen supplementation (hyperoxia; HO) is the most frequently administered therapy in hospitalized patients and the mainstay of treatment for hypoxic respiratory failure, regardless of its etiology 1 . Clinically, supra-physiologic levels of oxygen tension are often tolerated and perceived as a safety net against hypoxemia 2 . As a result, in the US each year approximately 800,000 patients are exposed to HO therapy at a cost of $1.8 billion to the health care budget 3 . Importantly, the degree and duration of HO exposure positively correlate with patient morbidity and mortality rates 4 , 5 , 6 .
Although oxygen therapy can be a life-saving intervention, ample experimental and clinical evidence demonstrates that excessive levels of oxygen supplementation can also initiate and accelerate existing lung injury (HO-induced acute lung injury; HALI). Animal models of HALI have been particularly helpful in investigating the underlying mechanisms 7 , and studies in healthy adults showed that HO exposure causes tracheobronchitis and changes in vital capacity, diffusing capacity, and lung permeability within only six hours, and with a severity that is proportional to the degree of HO exposure 8 , 9 , 10 , 11 , 12 , 13 . Experimentally, a similar dose- and time-dependent inflammatory response to HO can be reproduced in animal models of HALI 14 , 15 , 16 , demonstrating close similarities in lung injury phenotypes between animals and humans 15 , 17 , 18 , 19 , 20 . From these studies we learned that the molecular mechanisms underlying HALI are complex and include extensive alterations in inflammatory cytokine secretion 14 , 21 , 22 . Both alveolar epithelial and endothelial cells are injured by HO, but the epithelial layer is the first line of defense against inhaled HO 23 , 24 , 25 .
Currently, minimizing the duration and amount of HO exposure of patients (“permissive hypoxemia”) represents the only clinical approach to limit HALI, and so far no molecular targets have been identified that translate into improved patient outcomes 26 . However, minimizing HO exposure is complicated by the lack of consensus in defining the lower limits of permissive hypoxemia, which would allow us to clinically differentiate beneficial from injurious levels of HO therapy 27 , 28 .
In our search for new molecular targets against HALI, we recently identified epithelial K 2P 2.1 (TREK-1) K + channels as important regulators in the development and progression of HALI 29 , 30 , 31 , 32 . TREK-1 channels belong to the family of 2-pore domain (K2P) K + channels, which are generally known for their unusual gating properties leading to so-called “leak K + currents” that stabilize the resting plasma membrane potential (Em) 33 , 34 . In general, K2P channels, including TREK-1, are widely expressed in body tissues 35 , 36 , 37 , 38 , 39 , 40 , 41 , but their role in the lung remains largely unknown. Using in vivo and in vitro models of HALI, we previously discovered that HO exposure decreases the expression of TREK-1 channels in mouse lungs and alveolar epithelial cells, and accelerates alveolar inflammation and barrier dysfunction 30 , 42 , 43 . These findings sparked the hypothesis that despite HO-mediated TREK-1 downregulation, pharmacological activation of the remaining subset of TREK-1 channels can protect against HALI. To test this hypothesis, in this study we explored the potential protective effects and underlying mechanisms of two novel TREK-1 activating compounds (ML335, BL1249) using in vivo and in vitro models of HALI.
Intra-tracheal administration of TREK-1 activating compounds protects mice against HO-induced acute lung injury (HALI)
Building on our previous findings that HO downregulates TREK-1 expression 42 , we determined whether pharmacological activation of the remainder subset of TREK-1 channels can counteract the injurious effects of HO on mouse lungs. We treated WT mice with once-daily intra-tracheal ( i.t. ) injections of the TREK-1-activating compounds ML335 or BL1249 44 , 45 , or an equimolar drug vehicle control, for a total of 3 injections over the 72-h HO or room air (RA) exposure period. Histological analysis (Fig. 1 A) and blinded Lung Injury Scoring (LIS; Fig. 1 B) of H&E-stained mouse lung sections revealed that under RA conditions administration of ML335 and BL1249 had no damaging effect on lung histology. As expected, exposure of mice to HO caused significant inflammatory changes (panel d), as also evidenced by an increase in LIS. Importantly, once-daily i.t. injections of ML335 or BL1249 during HO exposure substantially reduced these HO-induced injurious effects (Fig. 1 A panels e and f, and B). Similarly, analysis of physiological parameters of lung injury also revealed protective effects of TREK-1 activation in HO-exposed mice, as evidenced by improvements in quasi-static lung compliance (Fig. 1 C), and a reduction in BAL fluid protein levels and total cell counts (Fig. 1 D, E). These data suggest that pharmacological activation of TREK-1 channels can counteract HALI in an experimental mouse model.
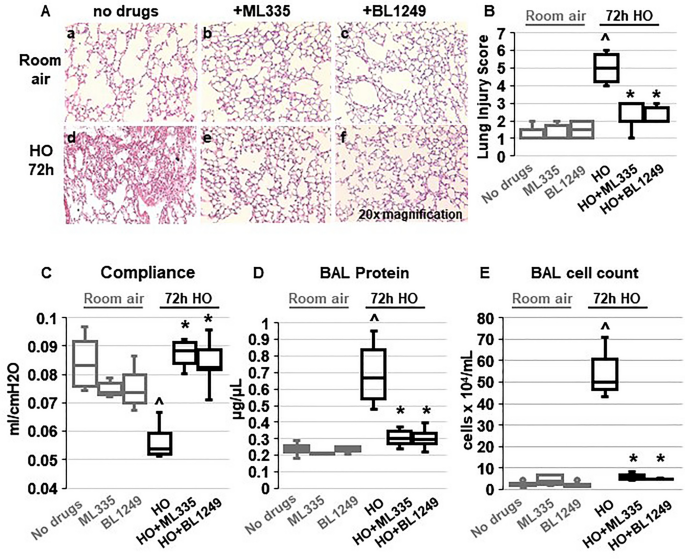
TREK-1 activation with the novel compounds ML335 and BL1249 protects form HO-induced lung injury: ( A ) Representative images of H&E-stained lung sections of WT mice exposed to either room air (panels a-c) or HO (panels d-f) for 72 h. All mice received once-daily, intra-tracheal ( i.t. ) injections of ML335, BL1249, or a vehicle control (no drugs) via brief endotracheal intubation. HO exposure caused significant lung injury (panel d), which was ameliorated by concomitant treatment with ML335 or BL1249 (panels e, f). ( B ) Summary of cumulative Lung Injury Scores of n = 5 independent experiments. ( C – E ) The HO-induced decrease in semi-static lung compliance, and increase in BAL fluid total protein and cell count were counteracted by ML335 and BL1249. Data are represented as Box-Whisker plots with medians, 1st and 3rd quartiles, and max and min values; n = 5–9; ^compared to mice injected with a vehicle control and exposed to room air (no drugs), *compared to HO exposed mice; p ≤ 0.05.
TREK-1 activation decreases inflammatory cytokine concentrations in the BAL fluid of HO exposed mice
To investigate whether the TREK-1-mediated improvements in histological and physiological lung injury parameters are associated with a reduction in inflammatory cytokine concentrations in HO exposed mice, we measured IL-6, IP-10, CCL-2, TNF-α, MIP-1α and IL-10 concentrations in BAL fluid (Fig. 2 ). We found that under room air conditions once-daily i.t. injections of ML335 or BL1249 had no effect on baseline cytokine secretion. Exposure of mice to 72 h HO resulted in an increase in IL-6, IP-10, CCL-2, TNF-α and IL-10 concentrations in the BAL fluid. Importantly, once-daily i.t. injections with ML335 or BL1249 during the 72 h of HO exposure decreased HO-induced IL-6 and IP-10 levels in the BAL fluid, but not CCL-2, TNF-α or IL-10. MIP-1α concentrations were neither affected by HO exposure nor treatment of mice with the TREK-1 activating compounds.
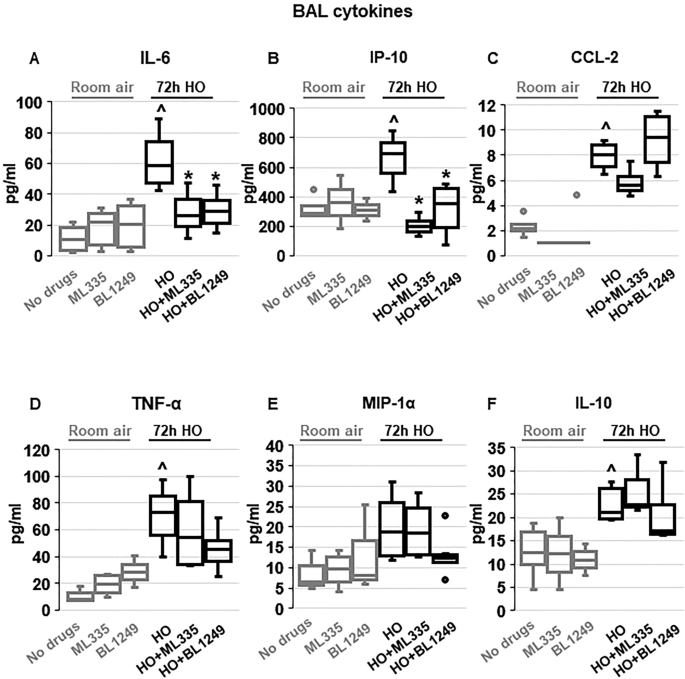
Effects of ML335 and BL1249 on BAL fluid cytokine concentrations ( A – F ): HO exposure increased IL-6, IP-10, CCL-2, TNF-α, and IL-10 concentrations, but not MIP-1α. Once-daily i.t . treatment with ML335 or BL1249 decreased HO-induced IL-6 and IP-10 levels, but not CCL-2, TNF-α or IL-10. Data are represented as Box-Whisker plots with medians, 1st and 3rd quartiles, and max and min values; n = 5–9; ^compared to mice exposed to room air and treated with a vehicle control (no drugs), *compared to HO exposed mice; p ≤ 0.05.
TREK-1 activity regulates inflammatory cytokine secretion from primary mouse AT2 cells
To evaluate whether the protective effects of TREK-1 activation observed in vivo were due to TREK-1-mediated attenuation of inflammatory cytokine secretion from alveolar epithelial cells, we exposed freshly-isolated mouse AT2 cells to HO or RA in the presence or absence of ML335 or BL1249, and quantified inflammatory cytokine secretion in culture supernatants (Fig. 3 ). We chose the shorter (24-h) HO exposure period (compared to 72 h in vivo) for freshly isolated AT2 cells, since in this cell type 72 h of HO exposure resulted in > 60% AT2 cell death (data not shown). Importantly, real-time PCR experiments and immunofluorescence (IF) microscopy imaging confirmed HO-induced TREK-1 downregulation after 24 h in this cell-type (Supplementary Fig. 1 A,B). Similar to our findings in the BAL fluid, HO exposure increased secretion of IL-6 and CCL-2 from freshly isolated mouse AT2 cells, and this effect was counteracted by concomitant treatment of cells with the TREK-1 activators ML335 or BL1249. Furthermore, HO exposure did not induce MIP-1α secretion from AT2 cells, similar to our findings in the BAL fluid. In contrast to our findings in the BAL fluid, HO exposure did not induce IP-10, TNF-α or IL-10 secretion from primary AT2 cells, and treatment with ML335 or BL1249 had no effect on the secretion of these cytokines at baseline or after HO exposure (Fig. 3 ).
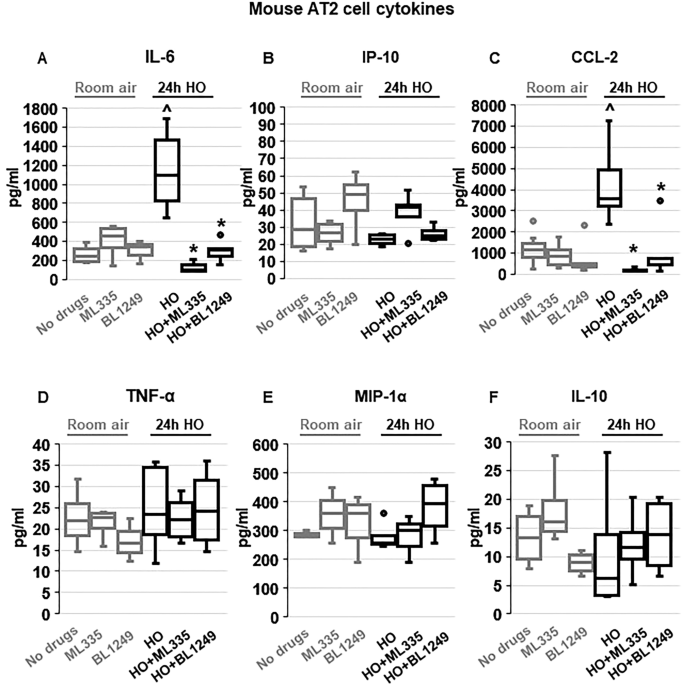
TREK-1 activation with ML335 and BL1249 regulates cytokine secretion from primary mouse AT2 cells: HO exposure increased IL-6 and CCL-2 secretion, which were inhibited by concomitant treatment with ML335 or BL1249 ( A , C ). In contrast, IP-10, TNF-α, MIP-1α and IL-10 levels were not affected by TREK-1 activation in room air- or HO-exposed AT2 cells ( B , D , E , F ). Data are represented as Box-Whisker plots with medians, 1st and 3rd quartiles, and max and min values; n = 5–9; ^compared to cells treated with a vehicle control and exposed to room air (no drugs), *compared to HO exposed cells; p ≤ 0.05.
TREK-1 activity regulates inflammatory cytokine secretion from primary human alveolar epithelial cells (HAECs)
To determine whether the TREK-1-mediated protective effects observed in mice and mouse AT2 cells can be reproduced in primary human alveolar epithelial cells (HAEC), we exposed HAEC to 72 h HO in the presence or absence of the TREK-1 activators ML335 or BL1249 (Fig. 4 ). Initial dose–response experiments revealed that BL1249 and ML335 are not cytotoxic at the doses used in this study (Supplementary Fig. 2 ). In contrast to primary mouse AT2 cells, viability of HAECs after 72 h HO exposure remained > 70% (data not shown), and this exposure period closely mimicked our in vivo model. Similar to our findings in primary mouse AT2 cells, HO exposure increased secretion of IL-6 and CCL-2 from HAECs, but did not induce TNF-α or MIP-1α secretion. Of note, overall concentrations of TNF-α and MIP-1α were quite low in these cells. In contrast to primary mouse AT2 cells but similar to BAL fluid, HO also increased secretion of IP-10 and IL-10 from HAECs. Importantly, treatment of HAECs with the TREK-1 activators ML335 or BL1249 inhibited the HO-induced secretion of IL-6, IP-10, CCL-2, and IL-10.
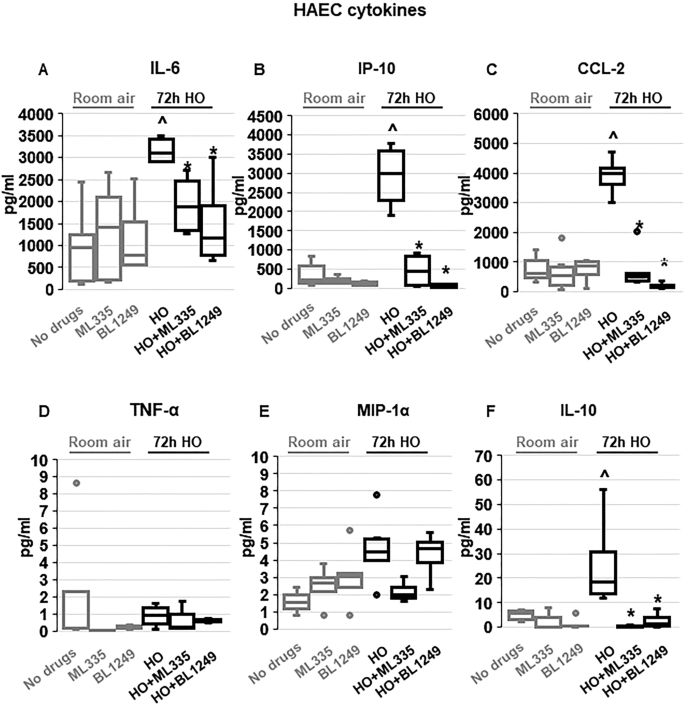
TREK-1 activation with ML335 and BL1249 regulates cytokine secretion from primary human alveolar epithelial cells (HAEC): HO exposure increased secretion of IL-6, IP-10, CCL-2 and IL-10, and this effect was counteracted by ML335 or BL1249 ( A , B , C , F ). In contrast, TNF-α and MIP-1α levels were not affected by TREK-1 activation in room air- or HO-exposed HAECs ( D , E ). Data are represented as Box-Whisker plots with medians, 1st and 3rd quartiles, and max and min values; n = 4–8; ^compared to cells treated with a vehicle control and exposed to room air (no drugs), *compared to HO exposed cells; p ≤ 0.05.
Altogether, these findings suggest that TREK-1 activation regulates HO-induced inflammatory cytokine secretion both in vivo and in vitro, but differences can be observed between overall cytokine concentrations in BAL fluid and cultured primary epithelial cells.
ML335 and BL1249 activate TREK-1 currents in primary AT2 cells
Although the specificity of ML335 and BL1249 for TREK-1 channels has previously been validated in heterologous expression systems 44 , 46 , 47 , the effectiveness of these compounds has never been demonstrated in lung cells. To confirm that both compounds activate TREK-1 currents in a physiologically relevant system and cell type, we used fluorescence-based, K + -sensitive FLIPR assays to demonstrate the effects of ML335 and BL1249 on K + currents in primary mouse AT2 cells (Fig. 5 ). FLIPR assays exploit the permeability of thallium (Tl + ) for open K + channels 48 . After loading AT2 cells with the fluorescent dye, the addition of extracellular Tl + creates a concentration gradient for Tl + to enter the cells. The resultant increase in relative fluorescence is proportional to the open probability of plasma membrane K + channels, and thus represents a measure of the functional activity of K + channels. Therefore, under unstimulated conditions (no drugs), the Tl + -induced fluorescence represents the sum of background K + currents, while after ML335 or BL1249 treatment an increase in fluorescence represents activation of TREK-1-specific K + currents (Fig. 5 ). Our data show that under RA conditions both compounds, ML335 and BL1249, activate TREK-1-specific K + currents (Fig. 5 A). Importantly, these effects were maintained after 24 h of HO exposure (Fig. 5 B).
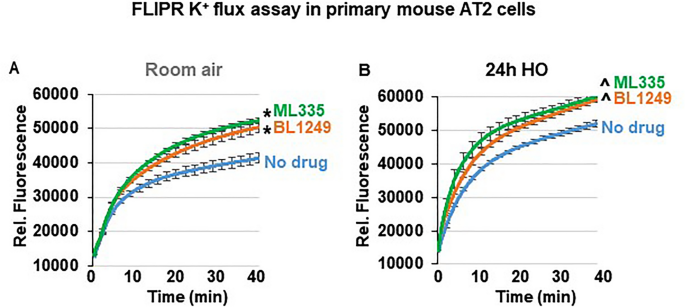
ML335 and BL1249 activate TREK-1 currents in primary mouse AT2 cells: Summary of n = 4–5 independent FLIPR curves (means ± SEM) showing that the TREK-1 activating compounds ML335 or BL1249 induce TREK-1 specific K + currents under both room air and HO conditions ( A , B ). In both room air and HO-treated cells, baseline background K + currents were observed (No drug). *compared to vehicle control (No drug) at room air, ^compared to vehicle control (No drug) after HO exposure; p ≤ 0.05, n = 4–5, individual experiments were run in triplicates.
Activation of TREK-1 channels hyperpolarizes the plasma membrane potential (Em) of primary AT2 cells
To determine whether the protective effects of ML335 and BL1249 are mediated by TREK-1-induced alterations in the Em, we performed Em-sensitive FLIPR assays on primary mouse AT2 cells under RA and HO conditions (Fig. 6 ). Once cells are loaded with the Em-sensitive fluorescent dye, a decrease in relative fluorescence represents Em hyperpolarization, whereas an increase in fluorescence represents Em depolarization. Since both TREK-1 activating compounds, ML335 and BL1249, had identical effects (i) in our in vivo model, (ii) on inflammatory cytokine secretion, and (iii) on TREK-1 current activation, we used only BL1249 for this part of the study. We found that under room air conditions, activation of TREK-1 channels with BL1249 results in Em hyperpolarization (= a decrease in fluorescence; Fig. 6 A) of primary AT2 cells. Similar to the observed activation of TREK-1 currents with BL1249 (Fig. 5 ), the BL1249-induced Em hyperpolarization also persisted after HO exposure (Fig. 6 B). Importantly, these studies also revealed that HO itself causes Em depolarization when compared to cells kept at RA, as evidenced by a higher baseline fluorescence value in HO-exposed cells (see RED arrows on the Y-axis → in Fig. 6 A, B and summarized in C).
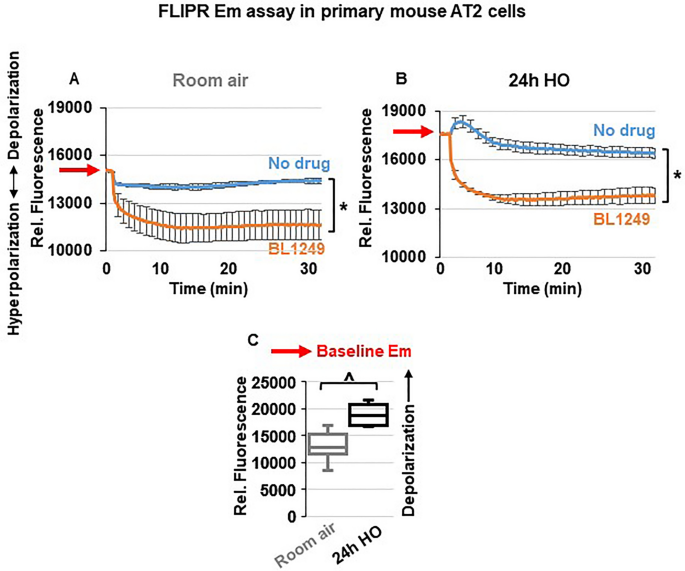
TREK-1 activation causes plasma membrane potential (Em) hyperpolarization: Representative curves of Em-sensitive FLIPR assays showing that TREK-1 activation with BL1249 causes Em hyperpolarization in primary mouse AT2 cells under both room air and HO conditions ( A , B ), as indicated by a decrease in fluorescence values. Red arrows on the Y-axis indicate relative fluorescence values reflective of the baseline Em value in room air and HO exposed AT2 cells, demonstrating that HO exposure itself causes Em depolarization (higher baseline fluorescence value in B than A; *BL1249 compared to no drug/vehicle control, p ≤ 0.05). ( C ) Summary of baseline Em values of RA- vs. HO-exposed AT2 cells averaging n = 6 independent experiments for each condition. Data are represented as Box-Whisker plots with medians, 1st and 3rd quartiles, and max and min values; ^compared to room air exposed AT2 cells; p ≤ 0.05, individual experiments were run in triplicates.
TREK-1 activation decreases intracellular Ca 2+ (iCa) levels during HO exposure
Since inflammatory cytokine secretion is commonly associated with an increase in iCa concentrations, we used Fluo-4 assays to determine the effects of TREK-1 activation on iCa levels in primary mouse AT2 cells (Fig. 7 ). Importantly, following 24 h of HO exposure, AT2 cells contained higher iCa concentrations than cells kept at RA (PURPLE arrows on the Y-axis → in Fig. 7 A,B and summarized in C). In cells kept at RA, activation of TREK-1 channels and Em hyperpolarization with BL1249 had no effect on iCa levels, likely due to the already low iCa levels in resting cells (Fig. 7 A). In HO-exposed cells, on the other hand, TREK-1 activation with BL1249 decreased the HO-induced elevation in iCa levels (Fig. 7 B).
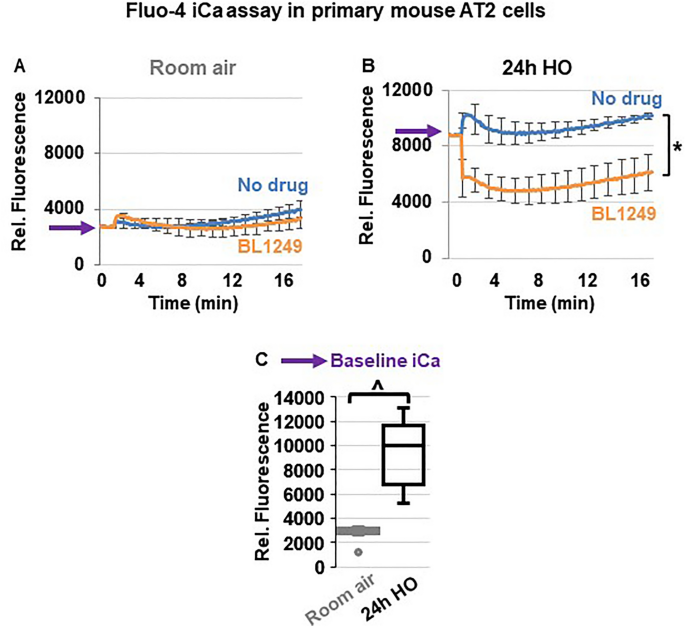
TREK-1 activation decreases intracellular Ca 2+ (iCa) concentrations in HO-exposed primary mouse AT2 cells: ( A , B ) Representative curves of Ca 2+ -sensitive Fluo-4 assays showing that HO-exposed AT2 cells contain higher iCa concentrations than RA-exposed cells, as indicated by an increase in fluorescence values (purple arrows on Y-axes). TREK-1 activation with BL1249 has no effect on iCa concentrations in RA-exposed cells, but decreases iCa levels in HO-exposed cells (*BL1249 compared to no drug/vehicle control, p ≤ 0.05). A summary of n = 6 independent experiments is shown in C ; data are represented as Box-Whisker plots with medians, 1st and 3rd quartiles, and max and min values; ^compared to room air exposed AT2 cells; p ≤ 0.05, individual experiments were run in triplicates.
Altogether, these findings highlight the TREK-1 activating effects and resultant Em hyperpolarization caused by ML335 and BL1249 in primary alveolar epithelial cells, and demonstrate that these effects persist under HO conditions, making TREK-1 activation a feasible approach to modulate the Em and iCa concentrations during HO exposure.
Regulation of inflammatory cytokine secretion by voltage-gated Ca 2+ (Ca V ) channels
Em depolarization, as observed with HO exposure and counteracted by TREK-1 activation, results in opening of Ca V channels in many cell types, and the resultant increase in iCa concentrations is commonly a trigger for downstream inflammatory cytokine secretion 49 , 50 . Therefore, we measured HO-induced cytokine secretion from primary mouse AT2 cells after blocking N- and P/Q-type Ca V channels with ω-conotoxin MVIIC 51 , and L-type Ca V channels with nifedipine 52 (Fig. 8 ). Since in primary AT2 cells HO exposure predominantly induced secretion of IL-6 and CCL-2 (Fig. 3 ), we focused on the role of Ca V channels in the secretion of these two cytokines. Interestingly, while HO-induced IL-6 secretion was not dependent on Ca V channel activity, CCL-2 secretion was inhibited by the L-type Ca V channel blocker nifedipine, but not by the N- and P/Q-type Ca V channel blocker ω-conotoxin MVIIC. Secretion of IP-10, TNF-α, MIP-1α and IL-10 from AT2 cells was not affected by ω-conotoxin MVIIC or nifedipine (data not shown).
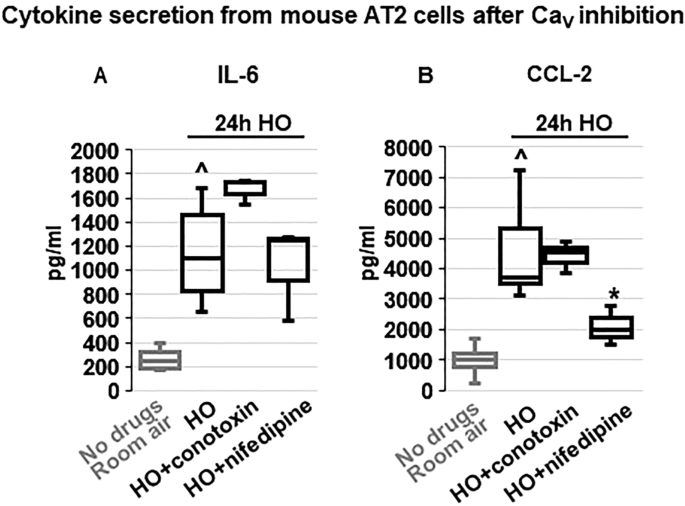
Effects of voltage-gated Ca 2+ channel (Ca V ) inhibition on cytokine secretion from primary mouse AT2 cells: HO exposure increased IL-6 ( A ) and CCL-2 ( B ) secretion compared to RA-treated cells. Inhibition of N- and P/Q-type Ca V channels with ω-conotoxin MVIIC or L-type Ca V channels with nifedipine revealed that IL-6 secretion occurred independently of Ca V channel activity, whereas CCL-2 secretion was dependent on L-type Ca V channels (inhibited by nifedipine) but not N- and P/Q-type channels (lack of ω-conotoxin MVIIC effect). Data are represented as Box-Whisker plots with medians, 1st and 3rd quartiles, and max and min values; n = 3–6; ^compared to cells treated with a vehicle control and exposed to room air (No drugs), *compared to HO exposed cells; p ≤ 0.05.
Altogether, these findings suggest that regulation of inflammatory cytokine secretion via TREK-1-induced Em hyperpolarization and inhibition of Ca V channel activation could explain some, but not all, of the TREK-1 protective effects seen in our in vivo model.
In this study we propose activation of TREK-1 K + channels as a potentially new therapeutic approach against HALI, since currently no targeted interventions exist that translate into improved patient outcomes. Recent in vitro studies suggest that overexpression of certain microRNAs (miR16, miR21-5) may protect cultured AT2 cells against HO-induced apoptosis 53 , 54 , 55 , and multiple biomarker studies have aimed at predicting the risk of HALI in patients 56 , 57 . In addition, neutralizing therapies against individual cytokines, including IL-6, TNF-α and CCL-2, have yielded variable results at best in improving inflammatory responses 58 , 59 , 60 , 61 .
Given these challenges, we are particularly interested in identifying strategies that can regulate multiple inflammatory pathways simultaneously, such as the manipulation of the plasma membrane potential (Em). We previously discovered that HO downregulates TREK-1 K + channel expression in lung tissue and alveolar epithelial cells, which correlates with worsening lung injury and alterations in multiple inflammatory cytokines (IL-6, CCL-2, RANTES, and IL-1β) 29 , 30 , 31 , 32 , 42 . Importantly, this HO-induced decrease in TREK-1 expression leaves a remainder subset of TREK-1 channels suitable for pharmacological activation. Although channels of the K2P family are known for their so-called “leak K + currents” (a constant, slow K + efflux that stabilizes the Em), TREK-1 channels are actually thought to be closed at baseline 34 , 62 . This idea is supported by our own data in alveolar epithelial cells (Fig. 5 ) showing that TREK-1 currents can readily be induced by our channel activators ML335 and BL1249 44 , 46 , 47 , thus making TREK-1 channels a feasible target for therapeutic activation.
So far, most of the biophysical characterization of TREK-1 channels has occurred under non-physiological conditions in heterologous expression systems 44 , 63 , 64 , and little is known about their functions in physiologically-relevant models. Our study is the first to (a) report the safety and efficacy of the novel TREK-1 activating compounds ML335 and BL1249 in an in vivo system, and (b) highlight the protective effects of TREK-1 activation in a lung injury model by measuring clinically relevant parameters. The only other reports suggesting a potentially protective role for TREK-1 activation used models of hypoxic-ischemic brain injury and atrial fibrillation/heart failure 65 , 66 , 67 . Interestingly, effects of single nucleotide polymorphisms (SNPs) in the human TREK-1 gene have been reported in the same two organs, and predict resistance to antidepressant medication 68 , and an increased risk for atrial tachycardias 69 . However, until now a similar protective effect for TREK-1 channels has not been reported in any other organ.
The importance of inflammatory mediators in the development and progression of HALI is well-established 14 , 70 , including the cytokines reported in this study: IL-6, IP-10, CCL-2, TNF-α, MIP-1α, and IL-10 17 , 21 . In general, IL-6, IP-10, TNF-α and MIP-1α are known for their proinflammatory properties, while CCL-2 can exert pro- 71 , 72 or anti-inflammatory 73 , 74 effects, and IL-10 is considered a predominantly anti-inflammatory cytokine 75 , 76 . More recently it has become increasingly clear that the inflammatory phenotypes observed in various lung injury models are determined by complex interactions between multiple cytokines. For example, despite the well-documented proinflammatory effects of IL-6 and its association with poor outcomes in ARDS patients 77 , IL-6 also induces anti-inflammatory IL-10 secretion as a counter-regulatory response 78 , and a recent study suggests that IL-6 protects mice from LPS- and mechanical ventilation-induced lung injury 79 . In our HALI model we found increased levels of both IL-6 and IL-10 in the BAL fluid of HO-exposed mice. Interestingly, while TREK-1 activation decreased HO-induced BAL fluid IL-6 levels, IL-10 levels remained elevated even after TREK-1 activation, potentially acting synergistically with the protective effects of TREK-1 activation. It is important to note that both lung resident and immune cells contribute to the cytokine levels measured in BAL fluid, and it is quite likely that in our in vivo model the TREK-1 activators affect cytokine secretion from multiple cell types. In this study we focused on epithelial cells since previously we did not find alterations in TNF-α release from TREK-1-deficient alveolar macrophages, and the single cell RNA-seq database LungGENS only reports low levels of TREK-1 postnatally in endothelial cells 42 , 80 .
Our results report for the first time (1) the expression of functional TREK-1 channels on primary mouse AT2 cells and human alveolar epithelial cells (HAEC), and (2) the effects of Em manipulation via TREK-1 channels on inflammatory cytokine secretion and iCa concentrations in a clinically relevant model of HALI. Since in clinical practice the timing of HO therapy is entirely under the control of the healthcare provider, administration of TREK-1 activators simultaneously with initiation of HO therapy is a clinically feasible approach. Of note, although in animal models the injurious effects of HO on previously healthy lungs have been extensively studied, in humans the exact degree and duration of HO exposure that results in symptomatic and clinically-relevant injury remains a matter of intense discussion 81 .
From studies in macrophages, neutrophils and mast cells, we learned that changes in the Em commonly precede secretory events 82 , 83 , but the molecular mechanisms regulating inflammatory cytokine secretion from lung resident cells remain incompletely understood. Furthermore, studies in lung endothelial cells, revealed that the resting Em can vary among cell phenotypes. Reported Em values in endothelial cells range from − 30 to − 60 mV 84 , 85 , and exposure of pulmonary artery endothelial cells to low oxygen concentrations (hypoxia) has been reported to cause Em depolarization 86 . Similar variations in Em depending on the cellular phenotype have also been documented in lung epithelial cells, including rat AT2 cells (− 30 mV) 87 , 88 , rabbit AT2 cells (− 60 mV) 89 , human bronchial epithelial cells (− 20 to − 45 mV) 90 , 91 , and nasal epithelial cells (− 15 to − 30 mV) 91 , 92 . Limited information form human ex vivo studies point towards Em values between − 15 and − 20 mV in bronchial epithelial cells 91 , 93 . Notably, other studies estimate the resting Em in AT2 cells as low as 0 to − 5 mV 94 , 95 . Despite these ranges in Em for lung resident cells, it is important to realize that the Em of epithelial and endothelial cells is much lower than the Em of excitable cells such as neurons and cardiomyocytes, in which the Em ranges between − 60 and − 90 mV 96 , 97 . Since these latter cell types are more hyperpolarized at baseline (i.e. more negative Em values), they require a much stronger depolarization stimulus for a biological response to occur, such as the opening of voltage-gated Ca 2+ (Ca V ) channels and subsequent Ca 2+ influx. In contrast, in the more depolarized epithelial and endothelial cells, a much smaller Em perturbation can reach the threshold for Ca V channel activation, and trigger downstream responses. Conversely, K + efflux, as caused by TREK-1 activation with BL1249 (Fig. 6 A), moves the Em away from this critical threshold towards more negative (hyperpolarized) Em values, and can counteract depolarization-induced cell activation processes.
HO-mediated depolarization events have been reported in mitochondrial membranes of pulmonary endothelial cells 98 , but our study is the first to show HO-induced Em depolarization in primary epithelial cells. Interestingly, in carotid body cells hypoxia, not hyperoxia, causes Em depolarization and increases iCa 2+ concentrations, while HO inhibits both of these processes 99 , 100 . In contrast to these studies, we demonstrate that primary epithelial cells respond to HO exposure by increasing iCa 2+ levels (Fig. 7 ), and we propose that this response is mediated by HO-induced Em depolarization that can be counteracted by TREK-1 activation (Figs. 6 , 7 ).
Interestingly, although it is well-known that both extracellular Ca 2+ influx and Ca 2+ release from intracellular stores can increase iCa 2+ levels, we found that in primary mouse AT2 cells only secretion of CCL-2, but not IL-6, IP-10, TNF-α, or MIP-1α, was dependent on Ca 2+ influx via Ca V channels (Fig. 8 ). The lack of effect of ω-conotoxin MVIIC on cytokine secretion suggests that Ca 2+ influx via N-, and P/Q-type Ca V channels is unlikely to contribute to these processes. In addition to the novelty and importance of our data, these findings also indicate that Ca 2+ release from intracellular stores is likely to be involved in the observed secretory processes.
Although upregulation of CCL-2 in bronchial and alveolar epithelial cells under inflammatory conditions is well-documented 101 , 102 , 103 , it remains a matter of intense discussion whether CCL-2 secretion in the lung is a Ca 2+ -dependent process, and may ultimately depend on the specific cell type and inflammatory environment. In both immortalized and primary lung epithelial cells, inhibition of Ca 2+ sensing, Ca 2+ influx, and iCa 2+ release all prevent CCL-2 secretion, and in some instances also IL-6 release 104 , 105 . Conversely, it is known that in immune cells CCL-2 itself can increase iCa 2+ concentrations 106 , demonstrating the complex interactions underlying CCL-2 secretion. One study showed that the chemotactic function of CCL-2 can occur in the absence of any changes in iCa 107 , and in an LPS-induced lung injury model inhibition of cellular Ca 2+ sensing receptors (CaSR) decreased IL-6 and TNF-α, but not CCL-2, concentrations in the serum and BAL fluid 104 .
Since in our model inhibition of Ca V channels decreased CCL-2 secretion but no other measured cytokines, we should consider the possibility that TREK-1-induced changes in Em could be directly sensed by a voltage-sensitive protein at the plasma membrane level. For this to occur, such a protein would need to contain one or more transmembrane segments with free charges that can induce a so-called “gating current” following an alteration in Em. Although membrane-bound voltage sensors are well-characterized in the brain and heart 108 , 109 , in the lung this important topic has yet to be explored.
We previously reported an important role for TREK-1 in HALI using a TREK-1-deficient mouse model 42 , which revealed a similar injurious phenotype as can be obtained with HO-induced TREK-1 downregulation 42 . In this study, we now shed some light on how TREK-1 may regulate downstream signaling cascades during HO exposure. Based on the current and our previous studies, we propose that the primary mechanism underlying the HO-mediated effects on TREK-1 signaling consists in a decrease in TREK gene and protein expression levels, rather than potential HO-mediated post-translational modifications of the TREK-1 protein structure. Of note, in HEK293 cells, posttranslational TREK-1 phosphorylation has been reported, and resulted in TREK-1 inhibition 110 . However, even if such changes occurred in the lung, they do not seem to interfere with the activation effects of BL1249 and ML335 on TREK-1 channels. Since BL1249 and ML335 are designed to bind and functionally activate wildtype TREK-1 channels, substantial HO-induced structural/posttranslational changes to the TREK-1 structure are unlikely the cause for our reported outcomes. In fact, one of the key findings of this study is that BL1249 and ML335 can activate TREK-1 channels and ameliorate injury despite any HO-induced changes in the intra- and extracellular cellular environments. Notably, we previously reported TREK-1 expression in both AT1 and AT2 cells from mouse lung slices, as well as mouse alveolar macrophages (AMs), but saw only weak TREK-1 staining in the mouse lung endothelium. Interestingly, in that study we also found that LPS-induced TNF-α release from mouse AMs appears to occur independently of TREK-1 31 , suggesting that epithelial TREK-1 channels are the primary target for BL1249 and ML335 in our HALI model.
In conclusion, we report for the first time the functional expression of TREK- 1K + channels on primary alveolar epithelial cells. We show that pharmacological activation of TREK-1 channels during HO exposure is a novel and clinically feasible approach to protect against HALI by reducing inflammatory cell recruitment and barrier dysfunction in the lungs, which may at least in part be mediated by inhibition of inflammatory cytokine secretion. However, additional studies are required to identify other potential effector mechanisms contributing to TREK-1-mediated protection, which should include ROS production, cell death pathways, and inflammasome activation.
Materials and methods
C57bl/6 wild-type (WT) mice aged 9–12 weeks were obtained from Jackson Laboratories ( www.jax.org ). Mice were housed in same-sex groups of up to 5 mice per cage and provided with food and water ad libitum. For experimental purposes, mice were age- and gender-matched as closely as possible.
Mouse hyperoxia (HO) exposure
Using a rodent HO chamber and a 5-L oxygen concentrator (DeVilbiss Healthcare, #525DS), we exposed mice to HO (F i O 2 = 0.8–0.9 inside the chamber) for 72 h in their native cages with free access to food and water. Temperature, humidity and oxygen concentrations were monitored continuously using commercially available sensors (AcuRite 00325A1 for temperature and humidity; Hudson-RCI5800 for oxygen concentrations). During HO exposure, mice lost less than 10% of weight and appeared overall healthy. No deaths were observed. Control mice were exposed to room air (RA) for the same time period in their native cages.
TREK-1 activating compounds
We used two novel TREK-1 activating compounds, ML335 and BL1249. ML335 has been synthesized and validated by our collaborator Dr. Minor at UCSF 44 , who provided this compound to us as gift. BL1249 has most recently become commercially available (Tocris) 45 . Stock solutions for ML335 (100 mM) and BL1249 (100 mM) were prepared in DMSO. For in vivo experiments, we used a final concentration of 100 μM ML335 and 200 μM BL1249 in sterile PBS. For in vitro experiments in primary cells, we used a final concentration of 100 μM (60 μg/kg) ML335 and 10 μM (100 μg/kg) BL1249 suspended in culture media. Vehicle controls for all experiments contained equimolar amounts of the DMSO.
Intra-tracheal injections
During the 72 h of RA or HO exposure, mice were injected once-daily intratracheally ( i.t. ) via brief endotracheal intubation with either 40μL of the TREK-1 activating compounds ML335, BL1249, or a vehicle control in sterile PBS. Briefly, for i.t. injections, mice underwent brief inhaled isofluorane (2–5%) anesthesia until they lost consciousness, and were then suspended by their incisors on a 3.0 silk suture mounted on a 45 degree-angled stand. The tongue was gently extracted from the mouth and moved to the side using blunt forceps in order to visualize the vocal cords. Using fiberoptic guidance, a 20-gauge angiocatheter was passed through the vocal cords into the subglottic area, and 40 μL of drug or vehicle control were injected with a micropipettor. Mice were then placed back into their native cages and allowed to recover under a warming lamp until fully awake. No perianesthetic deaths were associated with this procedure.
Quasi-static lung compliance measurements
Following RA or HO exposure, a tracheostomy was performed using an 18-gauge steel catheter under general ketamine/xylazine anesthesia (intraperitoneal, 10 mg/kg ketamine; 20 mg/kg xylazine). Quasi-static lung compliance was measured using the Flexivent system (SQIREC). Pressure–volume curves (P–V) were recorded, and each set of P–V curves was preceded by an inflation maneuver to total lung capacity to insure equal standard lung volumes for each experiment. Quasi-static lung compliance was calculated by fitting data derived from the P–V curves to the Salazar-Knowles equation as previously described 111 . Rectal temperatures were maintained in physiologic range using a heat lamp. All experiments were terminal.
Broncho-alveolar lavage (BAL) fluid collection and lung histology
Following Flexivent measurements, BAL fluid was collected from all mice using a 1 ml syringe attached to the tracheostomy catheter. Two wash-outs were performed with 1 ml PBS/0.6 mM EDTA for BAL protein and cell count determination, and 1 ml PBS/0.5% BSA for cytokine assays. All samples were immediately placed on ice. Total BAL protein concentrations were measured using the Bradford assay (BioRad), and total BAL cell counts were performed using a Diff-Quick stain (Fisher Scientific). Thereafter, lung tissue was harvested and processed for histological examination. Briefly, the lungs were gently retrograde perfused via the right ventricle with 10 ml ice-cold PBS to remove red blood cells. Lung tissue was then removed en bloc and immediately perfused and fixed in 4% formalin. Paraffin-embedded sections were cut into 4 µm thick tissues slices using a Microtome, and H&E-stained for histological analysis. Lung Injury Scores (LIS) were determined by an investigator blinded to the experimental conditions on H&E-stained lung sections as previously described, using the following 3 criteria: (1) interstitial and alveolar edema, (2) cellular infiltrate, and 3) parenchymal and perivascular hemorrhage. Each criterion was assigned a score between 0–3, with “0” representing no injury, “1” representing mild injury, “2” representing moderate injury, and “3” representing severe injury. Five randomly assigned high power fields per slide were scored under 40 × magnification on a Motic AE20/21 inverted microscope, and scores were averaged for each criterion. Using the sum of these averages, a composite histological LIS was calculated for each experimental group.
Primary mouse and human alveolar epithelial cells
Primary mouse alveolar type-2 cells (AT2) cells were freshly isolated as previously described 112 . We obtained in average 3–5 × 10 6 AT2 cells per mouse lung with > 90% purity as assessed by immunostaining for pro-SPC. All experiments were performed in accordance with the guidelines of the Institutional Animal Care and Use Committee at the University of California Los Angeles. Freshly isolated AT2 cells were seeded to 70–80% confluence at a density 3.5 × 10 6 cells per well in 6-well tissue culture plates coated with fibronectin. Cells were maintained in DMEM cell culture medium containing 10% FBS, 4 mM glutamine, 1% penicillin/streptomycin, and 0.25 µM amphotericin B. All experimental interventions were started on day 2 after AT2 cell isolation.
Primary Human Alveolar Epithelial Cells (HAEC) were purchased from ScienCell (#3200), cultured according to the company’s instructions, and used at a passage numbers < P5. Since these cell suspensions are directly isolated from donated human lung tissue, they contain mixed populations of AT1 and AT2 cells.
HO exposure of cells
HO exposure of cells was performed using a cell culture-compatible HO chamber. HAEC were exposed to 72 h of HO to mimic our in vivo HO protocol. Since in freshly isolated mouse AT2 cells we observed substantial cell death after 72 h of HO exposure (F i O 2 0.8–0.9), we limited HO exposure to 24 h for these cells. Controls for each cell type were cultured at room air for the respective time intervals. During the HO or RA exposure period, cell suspensions were treated with a one-time dose of the TREK-1 activating compounds ML335 (100 μM) or BL1249 (10 μM), or an equimolar DMSO vehicle control. Under all experimental conditions cell viability remained greater than 75% as determined by Trypan Blue staining. To assure that BL1249 and ML335 were not cytotoxic at the doses used, we performed dose–response experiments using two cell viability assays, CCK-8 (APExBIO) and XTT (Biotium).
TREK-1 gene and protein expression
We used real-time PCR and IF microscopy to confirm HO-induced TREK-1 downregulation after 24 h in freshly isolated mouse AT2 cells. Briefly, for PCR experiments total RNA was isolated using a Qiagen RNeasy Mini Kit (Hilden, Germany), 1 μg RNA was reverse transcribed with a High Capacity cDNA Reverse Transcription kit (Applied Biosystems), and amplified by semi-quantitative real-time PCR (TaqMan) with primers specific for TREK-1 (KCNK2; Applied Biosystems). For IF microscopy, mouse AT2 cells were fixed with 4% paraformaldehyde and then incubated with an anti-TREK-1 primary antibody (Alomone, 1:200) at 4 °C overnight, followed by probing with a species-specific secondary antibody (1:1000; Abcam) for one hour at room temperature. Nuclei were counterstained with Fluoro Gel II mounting medium containing DAPI (EMS). All images were recorded using Zen 2009 Light Edition software version 5.5 (Zeiss; https://www.zeiss.com/microscopy/us/products/microscope-software/zen-lite.html ).
Cytokine measurements by ELISA
Cytokine concentrations were quantified in BAL fluid and cell culture supernatants after centrifugation at 8000 rpm for 5 min. Briefly, 100 μL of sample was loaded into 96-well ELISA plate, and analyzed following the manufacturer’s instructions. All samples were run in triplicates and values are displayed in pg/mL. Species-specific ELISA kits were purchased from the following vendors: IL-6 (BD Biosciences), IP-10 (mouse: R&D Systems; human: BD Biosciences), CCL-2 (BD Biosciences), TNF-α (BD Biosciences), MIP-1α (R&D Systems), IL-10 (R&D Systems).
FLIPR and Fluo-4 assays for K + flux, plasma membrane potential (Em), and intracellular Ca 2+ (iCa) measurements
K + channel activity and Em measurements were performed using commercially available FLIPR assays (Molecular Devices, #R8222 and #R8126, respectively), and Fluo-4 assays (Invitrogen, #F36206) for iCa measurements. All three assays were performed following the manufacturer’s instructions. Briefly, for all assays 30,000 cells/well were seeded into dark-walled, clear-bottom 96-well plates (Grenier Bio-One, #655090), and cultured in growth medium overnight. The next day, cells were washed once and incubated at 37 °C with the respective loading dye for 60 min for K + channel activity assays, and 30 min for Em and Fluo-4 assays. In all assays, fluorescence traces were recorded for 1 min to reach a stable baseline before the addition of any drugs. All plates were analyzed using a BioTek Synergy-2 fluorescence plate reader. Data points were collected and integrated every 7 s. To determine whether an increase in iCa concentrations was due to Ca 2+ influx via voltage-gated Ca 2+ (Ca V ) channels, we blocked N- and P/Q-type Ca V channels with ω-conotoxin MVIIC (1 μM), and L-type Ca V channels with nifedipine (10 μM).
Statistical analysis
Quasi-static lung compliance, BAL protein and cell counts, LIS values, cytokine concentrations, and FLIPR and Fluo-4 data are represented as Box-Whisker plots with median values, 1st and 3rd quartiles, and maximum and minimum values. FLIPR curves in Figs. 5 A,B, 6 A,B, and 7 A,B show mean + SEM values. Data were analyzed using the unpaired student t-test, multivariate analysis of variance (ANOVA), and pairwise comparison of means using the Tukey–Kramer method to adjust for multiple comparisons. All statistical analyses were performed using GraphPad Prism 7 software (version 6.04, La Jolla, CA; https://www.graphpad.com/ ), and p values p ≤ 0.05 were considered significant.
Study approval
Approval for all experiments was obtained from the “University of California Los Angeles Animal Research Committee (ARC). All experiments were performed in accordance with our institutional protocols, guidelines and recommendations.
Scala, R. & Heunks, L. Highlights in acute respiratory failure. Eur. Respir. Rev. https://doi.org/10.1183/16000617.0008-2018 (2018).
Article PubMed Google Scholar
Suzuki, S., Eastwood, G. M., Peck, L., Glassford, N. J. & Bellomo, R. Current oxygen management in mechanically ventilated patients: A prospective observational cohort study. J. Crit. Care 28 , 647–654. https://doi.org/10.1016/j.jcrc.2013.03.010 (2013).
Kim, V., Benditt, J. O., Wise, R. A. & Sharafkhaneh, A. Oxygen therapy in chronic obstructive pulmonary disease. Proc. Am. Thorac. Soc. 5 , 513–518. https://doi.org/10.1513/pats.200708-124ET (2008).
Article PubMed PubMed Central Google Scholar
Girardis, M. et al. Effect of conservative vs conventional oxygen therapy on mortality among patients in an intensive care unit: The oxygen-ICU randomized clinical trial. JAMA https://doi.org/10.1001/jama.2016.11993 (2016).
Hale, K. E., Gavin, C. & O’Driscoll, B. R. Audit of oxygen use in emergency ambulances and in a hospital emergency department. Emerg. Med. J. 25 , 773–776. https://doi.org/10.1136/emj.2008.059287 (2008).
Article CAS PubMed Google Scholar
O’Driscoll, B. R., Howard, L. S., Bucknall, C., Welham, S. A. & Davison, A. G. British Thoracic Society emergency oxygen audits. Thorax 66 , 734–735. https://doi.org/10.1136/thoraxjnl-2011-200078 (2011).
Dias-Freitas, F., Metelo-Coimbra, C. & Roncon-Albuquerque, R. Jr. Molecular mechanisms underlying hyperoxia acute lung injury. Respir. Med. 119 , 23–28. https://doi.org/10.1016/j.rmed.2016.08.010 (2016).
Beckett, W. S. & Wong, N. D. Effect of normobaric hyperoxia on airways of normal subjects. J. Appl. Physiol. 1985 (64), 1683–1687. https://doi.org/10.1152/jappl.1988.64.4.1683 (1988).
Article Google Scholar
Caldwell, P. R., Lee, W. L. Jr., Schildkraut, H. S. & Archibald, E. R. Changes in lung volume, diffusing capacity, and blood gases in men breathing oxygen. J. Appl. Physiol. 21 , 1477–1483. https://doi.org/10.1152/jappl.1966.21.5.1477 (1966).
Davis, W. B., Rennard, S. I., Bitterman, P. B. & Crystal, R. G. Pulmonary oxygen toxicity. Early reversible changes in human alveolar structures induced by hyperoxia. N. Engl. J. Med. 309 , 878–883. https://doi.org/10.1056/nejm198310133091502 (1983).
Davis, W. B. et al. Pulmonary oxygen toxicity. Bronchoalveolar lavage demonstration of early parameters of alveolitis. Chest 83 , 35s (1983).
Article CAS Google Scholar
Dolezal, V. The effect of longlasting oxygen inhalation upon respiratory parameters in man. Physiol. Bohemoslov. 11 , 149–158 (1962).
CAS PubMed Google Scholar
Sackner, M. A., Landa, J., Hirsch, J. & Zapata, A. Pulmonary effects of oxygen breathing. A 6-hour study in normal men. Ann. Intern. Med. 82 , 40–43 (1975).
Helmerhorst, H. J. F. et al. Hyperoxia provokes a time- and dose-dependent inflammatory response in mechanically ventilated mice, irrespective of tidal volumes. Intensive Care Med. Exp. 5 , 27. https://doi.org/10.1186/s40635-017-0142-5 (2017).
de los Santos, R. et al. One hundred percent oxygen lung injury in adult baboons. Am. Rev. Respir. Dis. 136 , 657–661. https://doi.org/10.1164/ajrccm/136.3.657 (1987).
McElroy, M. C. et al. Nitric oxide attenuates lung endothelial injury caused by sublethal hyperoxia in rats. Am. J. Physiol. 272 , L631-638. https://doi.org/10.1152/ajplung.1997.272.4.L631 (1997).
Bhandari, V. Molecular mechanisms of hyperoxia-induced acute lung injury. Front. Biosci. 13 , 6653–6661 (2008).
Kallet, R. H. & Matthay, M. A. Hyperoxic acute lung injury. Respir. Care 58 , 123–141. https://doi.org/10.4187/respcare.01963 (2013).
Lv, R. et al. Advances in the therapy of hyperoxia-induced lung injury: Findings from animal models. Undersea Hyperb. Med. 41 , 183–202 (2014).
PubMed Google Scholar
Crapo, J. D. Morphologic changes in pulmonary oxygen toxicity. Annu. Rev. Physiol. 48 , 721–731. https://doi.org/10.1146/annurev.ph.48.030186.003445 (1986).
Bhandari, V. & Elias, J. A. Cytokines in tolerance to hyperoxia-induced injury in the developing and adult lung. Free Radic. Biol. Med. 41 , 4–18. https://doi.org/10.1016/j.freeradbiomed.2006.01.027 (2006).
Lee, P. J. & Choi, A. M. Pathways of cell signaling in hyperoxia. Free Radic. Biol. Med. 35 , 341–350 (2003).
Kondrikov, D., Gross, C., Black, S. M. & Su, Y. Novel peptide for attenuation of hyperoxia-induced disruption of lung endothelial barrier and pulmonary edema via modulating peroxynitrite formation. J. Biol. Chem. 289 , 33355–33363. https://doi.org/10.1074/jbc.M114.585356 (2014).
Article CAS PubMed PubMed Central Google Scholar
Pagano, A. & Barazzone-Argiroffo, C. Alveolar cell death in hyperoxia-induced lung injury. Ann. N. Y. Acad. Sci. 1010 , 405–416 (2003).
Article ADS CAS Google Scholar
Roper, J. M. et al. In vivo exposure to hyperoxia induces DNA damage in a population of alveolar type II epithelial cells. Am. J. Physiol. Lung Cell. Mol. Physiol. 286 , L1045-1054. https://doi.org/10.1152/ajplung.00376.2003 (2004).
Article ADS CAS PubMed Google Scholar
Abdelsalam, M. & Cheifetz, I. M. Goal-directed therapy for severely hypoxic patients with acute respiratory distress syndrome: Permissive hypoxemia. Respir. Care 55 , 1483–1490 (2010).
Tasker, R. C. Hyperoxemia and death of critically ill: Is there a problem of confounding by indication or outcome?. Am. J. Respir. Crit. Care Med. https://doi.org/10.1164/rccm.201909-1860LE (2019).
Barrot, L. et al. Liberal or conservative oxygen therapy for acute respiratory distress syndrome. N. Engl. J. Med. 382 , 999–1008. https://doi.org/10.1056/NEJMoa1916431 (2020).
Roan, E., Waters, C. M., Teng, B., Ghosh, M. & Schwingshackl, A. The 2-pore domain potassium channel TREK-1 regulates stretch-induced detachment of alveolar epithelial cells. PLoS ONE 9 , e89429. https://doi.org/10.1371/journal.pone.0089429 (2014).
Article ADS CAS PubMed PubMed Central Google Scholar
Schwingshackl, A., Teng, B., Ghosh, M. & Waters, C. M. Regulation of monocyte chemotactic protein-1 secretion by the two-pore-domain potassium (K2P) channel TREK-1 in human alveolar epithelial cells. Am. J. Transl. Res. 5 , 530–542 (2013).
CAS PubMed PubMed Central Google Scholar
Schwingshackl, A. et al. Regulation and function of the two-pore-domain (K2P) potassium channel Trek-1 in alveolar epithelial cells. Am. J. Physiol. Lung Cell. Mol. Physiol. 302 , L93–L102. https://doi.org/10.1152/ajplung.00078.2011 (2012).
Schwingshackl, A. et al. Regulation of interleukin-6 secretion by the two-pore-domain potassium (K2P) channel Trek-1 in alveolar epithelial cells. Am. J. Physiol. Lung Cell. Mol. Physiol. https://doi.org/10.1152/ajplung.00299.2012 (2012).
Honore, E. The neuronal background K2P channels: Focus on TREK1. Nat. Rev. Neurosci. 8 , 251–261. https://doi.org/10.1038/nrn2117 (2007).
Renigunta, V., Schlichthorl, G. & Daut, J. Much more than a leak: Structure and function of K(2)p-channels. Pflugers Arch. 467 , 867–894. https://doi.org/10.1007/s00424-015-1703-7 (2015).
Alloui, A. et al. TREK-1, a K+ channel involved in polymodal pain perception. EMBO J. 25 , 2368–2376. https://doi.org/10.1038/sj.emboj.7601116 (2006).
Xian Tao, L. et al. The stretch-activated potassium channel TREK-1 in rat cardiac ventricular muscle. Cardiovasc. Res. 69 , 86–97. https://doi.org/10.1016/j.cardiores.2005.08.018 (2006).
Tomuschat, C. et al. Altered expression of a two-pore domain (K2P) mechano-gated potassium channel TREK-1 in Hirschsprung’s disease. Pediatr. Res. 80 , 729–733. https://doi.org/10.1038/pr.2016.140 (2016).
Hivelin, C. et al. Potentiation of calcium influx and insulin secretion in pancreatic beta cell by the specific TREK-1 blocker spadin. J. Diabetes Res. 2016 , 3142175. https://doi.org/10.1155/2016/3142175 (2016).
Reyes, R. et al. Cloning and expression of a novel pH-sensitive two pore domain K+ channel from human kidney. J. Biol. Chem. 273 , 30863–30869 (1998).
Davis, K. A. & Cowley, E. A. Two-pore-domain potassium channels support anion secretion from human airway Calu-3 epithelial cells. Pflugers Arch. 451 , 631–641. https://doi.org/10.1007/s00424-005-1505-4 (2006).
Lembrechts, R. et al. Expression of mechanogated two-pore domain potassium channels in mouse lungs: Special reference to mechanosensory airway receptors. Histochem. Cell Biol. 136 , 371–385. https://doi.org/10.1007/s00418-011-0837-8 (2011).
Schwingshackl, A. et al. Deficiency of the two-pore-domain potassium channel TREK-1 promotes hyperoxia-induced lung injury. Crit. Care Med. 42 , e692-701. https://doi.org/10.1097/ccm.0000000000000603 (2014).
Schwingshackl, A. et al. Hyperoxia treatment of TREK-1/TREK-2/TRAAK-deficient mice is associated with a reduction in surfactant proteins. Am. J. Physiol. Lung Cell. Mol. Physiol. 313 , L1030-l1046. https://doi.org/10.1152/ajplung.00121.2017 (2017).
Lolicato, M. et al. K2P2.1 (TREK-1)-activator complexes reveal a cryptic selectivity filter binding site. Nature 547 , 364–368. https://doi.org/10.1038/nature22988 (2017).
Tertyshnikova, S. et al. BL-1249 [(5,6,7,8-tetrahydro-naphthalen-1-yl)-[2-(1H-tetrazol-5-yl)-phenyl]-amine]: A putative potassium channel opener with bladder-relaxant properties. J. Pharmacol. Exp. Therap. 313 , 250–259. https://doi.org/10.1124/jpet.104.078592 (2005).
Veale, E. L. et al. Influence of the N terminus on the biophysical properties and pharmacology of TREK1 potassium channels. Mol. Pharmacol. 85 , 671–681. https://doi.org/10.1124/mol.113.091199 (2014).
Pope, L. et al. Protein and chemical determinants of BL-1249 action and selectivity for K2P channels. ACS Chem. Neurosci. 9 , 3153–3165. https://doi.org/10.1021/acschemneuro.8b00337 (2018).
Zhang, D., Gopalakrishnan, S. M., Freiberg, G. & Surowy, C. S. A thallium transport FLIPR-based assay for the identification of KCC2-positive modulators. J. Biomol. Screen 15 , 177–184. https://doi.org/10.1177/1087057109355708 (2010).
Wirtz, H. R. & Dobbs, L. G. Calcium mobilization and exocytosis after one mechanical stretch of lung epithelial cells. Science (New York, N. Y.) 250 , 1266–1269 (1990).
Logan, M. R., Odemuyiwa, S. O. & Moqbel, R. Understanding exocytosis in immune and inflammatory cells: The molecular basis of mediator secretion. J. Allergy Clin. Immunol. 111 , 923–932 (2003) ( quiz 933 ).
Ramírez, D., Gonzalez, W., Fissore, R. A. & Carvacho, I. Conotoxins as tools to understand the physiological function of voltage-gated calcium (Ca(V)) channels. Mar. Drugs https://doi.org/10.3390/md15100313 (2017).
Hansen, P. B. et al. Functional importance of L- and P/Q-type voltage-gated calcium channels in human renal vasculature. Hypertension 58 , 464–470. https://doi.org/10.1161/hypertensionaha.111.170845 (2011).
Li, Z. et al. miR-16 inhibits hyperoxia-induced cell apoptosis in human alveolar epithelial cells. Mol. Med. Rep. 17 , 5950–5957. https://doi.org/10.3892/mmr.2018.8636 (2018).
Qin, S. et al. miR215p regulates type II alveolar epithelial cell apoptosis in hyperoxic acute lung injury. Mol. Med. Rep. 17 , 5796–5804. https://doi.org/10.3892/mmr.2018.8560 (2018).
Tamarapu Parthasarathy, P. et al. MicroRNA 16 modulates epithelial sodium channel in human alveolar epithelial cells. Biochem. Biophys. Res. Commun. 426 , 203–208. https://doi.org/10.1016/j.bbrc.2012.08.063 (2012).
Baumann, P. et al. Mechanical ventilation strategies alter cardiovascular biomarkers in an infant rat model. Physiol. Rep. https://doi.org/10.14814/phy2.13553 (2018).
Zinter, M. S. et al. Early plasma matrix metalloproteinase profiles: A novel pathway in pediatric acute respiratory distress syndrome. Am. J. Respir. Crit. Care Med. https://doi.org/10.1164/rccm.201804-0678OC (2018).
Bhargava, R. et al. Intratracheal IL-6 protects against lung inflammation in direct, but not indirect, causes of acute lung injury in mice. PLoS ONE 8 , e61405. https://doi.org/10.1371/journal.pone.0061405 (2013).
Minutti, C. M. et al. Local amplifiers of IL-4Ralpha-mediated macrophage activation promote repair in lung and liver. Science (New York, N. Y.) 356 , 1076–1080. https://doi.org/10.1126/science.aaj2067 (2017).
O’Byrne, P. M. The demise of anti IL-5 for asthma, or not. Am. J. Respir. Crit. Care Med. 176 , 1059–1060. https://doi.org/10.1164/rccm.200708-1264ED (2007).
Bagnasco, D., Ferrando, M., Varricchi, G., Passalacqua, G. & Canonica, G. W. A critical evaluation of anti-IL-13 and anti-IL-4 strategies in severe asthma. Int. Arch. Allergy Immunol. 170 , 122–131. https://doi.org/10.1159/000447692 (2016).
MacKenzie, G., Franks, N. P. & Brickley, S. G. Two-pore domain potassium channels enable action potential generation in the absence of voltage-gated potassium channels. Pflugers Arch. 467 , 989–999. https://doi.org/10.1007/s00424-014-1660-6 (2015).
Wiedmann, F. et al. N-glycosylation of TREK-1/hK(2P)2.1 two-pore-domain potassium (K(2P)) channels. Int. J. Mol. Sci. https://doi.org/10.3390/ijms20205193 (2019).
Braun, G., Lengyel, M., Enyedi, P. & Czirjak, G. Differential sensitivity of TREK-1, TREK-2 and TRAAK background potassium channels to the polycationic dye ruthenium red. Br. J. Pharmacol. 172 , 1728–1738. https://doi.org/10.1111/bph.13019 (2015).
Maingret, F., Patel, A. J., Lesage, F., Lazdunski, M. & Honore, E. Mechano- or acid stimulation, two interactive modes of activation of the TREK-1 potassium channel. J. Biol. Chem. 274 , 26691–26696 (1999).
Kim, D. Fatty acid-sensitive two-pore domain K+ channels. Trends Pharmacol. Sci. 24 , 648–654. https://doi.org/10.1016/j.tips.2003.10.008 (2003).
Schmidt, C. et al. Stretch-activated two-pore-domain (K2P) potassium channels in the heart: Focus on atrial fibrillation and heart failure. Prog. Biophys. Mol. Biol. 130 , 233–243. https://doi.org/10.1016/j.pbiomolbio.2017.05.004 (2017).
Perlis, R. H. et al. Pharmacogenetic analysis of genes implicated in rodent models of antidepressant response: Association of TREK1 and treatment resistance in the STAR(*)D study. Neuropsychopharmacology 33 , 2810–2819. https://doi.org/10.1038/npp.2008.6 (2008).
Decher, N. et al. Sodium permeable and “hypersensitive” TREK-1 channels cause ventricular tachycardia. EMBO Mol. Med. 9 , 403–414. https://doi.org/10.15252/emmm.201606690 (2017).
Meduri, G. U. et al. Persistent elevation of inflammatory cytokines predicts a poor outcome in ARDS plasma IL-1 beta and IL-6 levels are consistent and efficient predictors of outcome over time. Chest 107 , 1062–1073 (1995).
van Zoelen, M. A. et al. Endogenous MCP-1 promotes lung inflammation induced by LPS and LTA. Mol. Immunol. 48 , 1468–1476. https://doi.org/10.1016/j.molimm.2011.04.001 (2011).
Mercer, P. F. et al. Pulmonary epithelium is a prominent source of proteinase-activated receptor-1-inducible CCL2 in pulmonary fibrosis. Am. J. Respir. Crit. Care Med. 179 , 414–425. https://doi.org/10.1164/rccm.200712-1827OC (2009).
Zisman, D. A. et al. MCP-1 protects mice in lethal endotoxemia. J. Clin. Invest. 99 , 2832–2836. https://doi.org/10.1172/jci119475 (1997).
Narasaraju, T., Ng, H. H., Phoon, M. C. & Chow, V. T. MCP-1 antibody treatment enhances damage and impedes repair of the alveolar epithelium in influenza pneumonitis. Am. J. Respir. Cell Mol. Biol. 42 , 732–743. https://doi.org/10.1165/rcmb.2008-0423OC (2010).
Van Der Wal, S. et al. Lidocaine increases the anti-inflammatory cytokine IL-10 following mechanical ventilation in healthy mice. Acta Anaesthesiol. Scand. 59 , 47–55. https://doi.org/10.1111/aas.12417 (2015).
Kurosaki, F. et al. AAV6-mediated IL-10 expression in the lung ameliorates bleomycin-induced pulmonary fibrosis in mice. Hum. Gene Ther. 29 , 1242–1251. https://doi.org/10.1089/hum.2018.024 (2018).
Swaroopa, D. et al. Association of serum interleukin-6, interleukin-8, and acute physiology and chronic health evaluation II score with clinical outcome in patients with acute respiratory distress syndrome. Indian J. Crit. Care Med. 20 , 518–525. https://doi.org/10.4103/0972-5229.190369 (2016).
Andres-Hernando, A. et al. Circulating IL-6 upregulates IL-10 production in splenic CD4(+) T cells and limits acute kidney injury-induced lung inflammation. Kidney Int. 91 , 1057–1069. https://doi.org/10.1016/j.kint.2016.12.014 (2017).
Voiriot, G. et al. Interleukin-6 displays lung anti-inflammatory properties and exerts protective hemodynamic effects in a double-hit murine acute lung injury. Respir. Res. 18 , 64. https://doi.org/10.1186/s12931-017-0553-6 (2017).
Du, Y., Guo, M., Whitsett, J. A. & Xu, Y. ‘LungGENS’: A web-based tool for mapping single-cell gene expression in the developing lung. Thorax 70 , 1092–1094. https://doi.org/10.1136/thoraxjnl-2015-207035 (2015).
Hafner, S., Beloncle, F., Koch, A., Radermacher, P. & Asfar, P. Hyperoxia in intensive care, emergency, and peri-operative medicine: Dr. Jekyll or Mr. Hyde? A 2015 update. Ann. Intensive Care 5 , 42. https://doi.org/10.1186/s13613-015-0084-6 (2015).
Janiszewski, J., Huizinga, J. D. & Blennerhassett, M. G. Mast cell ionic channels: Significance for stimulus-secretion coupling. Can. J. Physiol. Pharmacol. 70 , 1–7 (1992).
Liu, D., Zhang, J., Wu, J., Zhang, C. & Xu, T. Altered calcium-induced exocytosis in neutrophils from allergic patients. Int. Arch. Allergy Immunol. 134 , 281–287. https://doi.org/10.1159/000079165 (2004).
Zhang, R. Z., Yang, Q., Yim, A. P. & He, G. W. Alteration of cellular electrophysiologic properties in porcine pulmonary microcirculation after preservation with University of Wisconsin and Euro-Collins solutions. Ann. Thorac. Surgery 77 , 1944–1950. https://doi.org/10.1016/j.athoracsur.2003.11.051 (2004).
Herskovic, J. J., Speyer, C. L., Simples, J. E., Steffes, C. P. & Ram, J. L. Lipopolysaccharide (LPS) enhancement of outward current in lung pericytes. Lung 180 , 215–220. https://doi.org/10.1007/s004080000095 (2002).
Zharikov, S. I., Herrera, H. & Block, E. R. Role of membrane potential in hypoxic inhibition of L-arginine uptake by lung endothelial cells. Am. J. Physiol. 272 , L78-84. https://doi.org/10.1152/ajplung.1997.272.1.L78 (1997).
Lee, S. Y., Maniak, P. J., Ingbar, D. H. & O’Grady, S. M. Adult alveolar epithelial cells express multiple subtypes of voltage-gated K+ channels that are located in apical membrane. Am. J. Physiol. Cell Physiol. 284 , C1614-1624. https://doi.org/10.1152/ajpcell.00429.2002 (2003).
Castranova, V., Jones, G. S. & Miles, P. R. Transmembrane potential of isolated rat alveolar type II cells. J. Appl. Physiol. Respir. Environ. Exerc. Physiol. 54 , 1511–1517. https://doi.org/10.1152/jappl.1983.54.6.1511 (1983).
Gallo, R. L., Finkelstein, J. N. & Notter, R. H. Characterization of the plasma and mitochondrial membrane potentials of alveolar type II cells by the use of ionic probes. Biochem. Biophys. Acta. 771 , 217–227. https://doi.org/10.1016/0005-2736(84)90536-4 (1984).
Falkenberg, C. V. & Jakobsson, E. A biophysical model for integration of electrical, osmotic, and pH regulation in the human bronchial epithelium. Biophys. J. 98 , 1476–1485. https://doi.org/10.1016/j.bpj.2009.11.045 (2010).
Knowles, M., Gatzy, J. & Boucher, R. Increased bioelectric potential difference across respiratory epithelia in cystic fibrosis. N. Engl. J. Med. 305 , 1489–1495. https://doi.org/10.1056/nejm198112173052502 (1981).
Gaillard, E. A., Shaw, N. J., Wallace, H. L., Vince, G. & Southern, K. W. Electrical potential difference across the nasal epithelium is reduced in premature infants with chronic lung disease but is not associated with lower airway inflammation. Pediatr. Res. 61 , 77–82. https://doi.org/10.1203/01.pdr.0000250035.10339.ce (2007).
Ballke, E. H., Wiersbitzky, S., König, A. & Jährig, K. Transepithelial potential difference in the bronchial system of children with chronic bronchitis and bronchial asthma. Padiatr. Grenzgeb. 31 , 103–105 (1992).
Shlyonsky, V., Goolaerts, A., Mies, F. & Naeije, R. Electrophysiological characterization of rat type II pneumocytes in situ. Am. J. Respir. Cell Mol. Biol. 39 , 36–44. https://doi.org/10.1165/rcmb.2007-0227OC (2008).
Canella, R. et al. Involvement of the TREK-1 channel in human alveolar cell membrane potential and its regulation by inhibitors of the chloride current. J. Cell. Physiol. 234 , 17704–17713. https://doi.org/10.1002/jcp.28396 (2019).
Crago, P. E. & Makowski, N. S. Alteration of neural action potential patterns by axonal stimulation: The importance of stimulus location. J. Neural Eng. 11 , 056016. https://doi.org/10.1088/1741-2560/11/5/056016 (2014).
Article ADS PubMed PubMed Central Google Scholar
Fozzard, H. A. & Sheu, S. S. The resting potential in heart muscle. Adv. Myocardiol. 3 , 125–133. https://doi.org/10.1007/978-1-4899-5561-6_14 (1982).
Ma, C. et al. Hyperoxia causes mitochondrial fragmentation in pulmonary endothelial cells by increasing expression of pro-fission proteins. Arterioscler. Thromb. Vasc. Biol. 38 , 622–635. https://doi.org/10.1161/atvbaha.117.310605 (2018).
Kim, I., Donnelly, D. F. & Carroll, J. L. Postnatal hyperoxia impairs acute oxygen sensing of rat glomus cells by reduced membrane depolarization. Adv. Exp. Med. Biol. 758 , 49–54. https://doi.org/10.1007/978-94-007-4584-1_7 (2012).
Kim, I., Yang, D., Carroll, J. L. & Donnelly, D. F. Perinatal hyperoxia exposure impairs hypoxia-induced depolarization in rat carotid body glomus cells. Respir. Physiol. Neurobiol. 188 , 9–14. https://doi.org/10.1016/j.resp.2013.04.016 (2013).
Sousa, A. R. et al. Increased expression of the monocyte chemoattractant protein-1 in bronchial tissue from asthmatic subjects. Am. J. Respir. Cell Mol. Biol. 10 , 142–147. https://doi.org/10.1165/ajrcmb.10.2.8110469 (1994).
Paine, R. III. et al. MCP-1 expression by rat type II alveolar epithelial cells in primary culture. J. Immunol. 150 , 4561–4570 (1993).
Becker, S., Quay, J., Koren, H. S. & Haskill, J. S. Constitutive and stimulated MCP-1, GRO alpha, beta, and gamma expression in human airway epithelium and bronchoalveolar macrophages. Am. J. Physiol. 266 , L278-286. https://doi.org/10.1152/ajplung.1994.266.3.L278 (1994).
Lee, J. W. et al. NPS 2143, a selective calcium-sensing receptor antagonist inhibits lipopolysaccharide-induced pulmonary inflammation. Mol. Immunol. 90 , 150–157. https://doi.org/10.1016/j.molimm.2017.07.012 (2017).
Cordazzo, C. et al. Rapid shedding of proinflammatory microparticles by human mononuclear cells exposed to cigarette smoke is dependent on Ca 2+ mobilization. Inflam. Res. 63 , 539–547. https://doi.org/10.1007/s00011-014-0723-7 (2014).
Proost, P., Wuyts, A. & Van Damme, J. Human monocyte chemotactic proteins-2 and -3: Structural and functional comparison with MCP-1. J. Leukoc. Biol. 59 , 67–74. https://doi.org/10.1002/jlb.59.1.67 (1996).
Gavrilin, M. A. et al. Site-directed mutagenesis of CCR2 identified amino acid residues in transmembrane helices 1, 2, and 7 important for MCP-1 binding and biological functions. Biochem. Biophys. Res. Commun. 327 , 533–540. https://doi.org/10.1016/j.bbrc.2004.12.037 (2005).
Bezanilla, F. How membrane proteins sense voltage. Nat. Rev. Mol. Cell Biol. 9 , 323–332. https://doi.org/10.1038/nrm2376 (2008).
Catterall, W. A. Ion channel voltage sensors: Structure, function, and pathophysiology. Neuron 67 , 915–928. https://doi.org/10.1016/j.neuron.2010.08.021 (2010).
Murbartian, J., Lei, Q., Sando, J. J. & Bayliss, D. A. Sequential phosphorylation mediates receptor- and kinase-induced inhibition of TREK-1 background potassium channels. J. Biol. Chem. 280 , 30175–30184. https://doi.org/10.1074/jbc.M503862200 (2005).
Salazar, E. & Knowles, J. H. An alaysis of pressure-volume characteristics of the lungs. J. Appl. Physiol. 19 , 97–104 (1964).
Messier, E. M., Mason, R. J. & Kosmider, B. Efficient and rapid isolation and purification of mouse alveolar type II epithelial cells. Exp. Lung Res. 38 , 363–373. https://doi.org/10.3109/01902148.2012.713077 (2012).
Download references
Acknowledgements
We thank Dr. Michela Ottolia (UCLA) and her lab for ongoing discussions and their intellectual input. We also thank Dr. Daniel Minor (UCSF) for providing us with the ML335 compound and for sharing with us his knowledge about the pharmacokinetics and pharmacodynamics of the compound. This study was supported by the following Grants: NIH HL118118-3 (AS); NIH HL131526 (CMW); NIH HL134346 (RO).
Author information
Authors and affiliations.
Department of Pediatrics, University of California Los Angeles, 10833 Le Conte Ave, MDCC 12-475, Los Angeles, CA, 90095, USA
Tatiana Zyrianova, Benjamin Lopez, Leanne Wong, Victoria Nguyen, Sriharsha Talapaneni & Andreas Schwingshackl
Department of Anesthesiology and Perioperative Medicine, University of California Los Angeles, Los Angeles, CA, USA
Riccardo Olcese
Department of Physiology, University of California Los Angeles, Los Angeles, CA, USA
Department of Pulmonary and Critical Care Medicine, University of California Los Angeles, Los Angeles, CA, USA
John Belperio
Department of Physiology, University of Kentucky, Lexington, KY, USA
Christopher M. Waters
You can also search for this author in PubMed Google Scholar
Contributions
T.Z.: experimental design and execution, manuscript writing and editing. B.L.: experimental design and execution. R.O.: experimental design and manuscript editing. J.B.: experimental design and manuscript editing. C.M.W.: experimental design and manuscript editing. L.W.: experimental execution. V.N.: experimental execution. S.T.: experimental execution. A.S.: experimental design, manuscript writing and editing.
Corresponding author
Correspondence to Andreas Schwingshackl .
Ethics declarations
Competing interests.
The authors declare no competing interests.
Additional information
Publisher's note.
Springer Nature remains neutral with regard to jurisdictional claims in published maps and institutional affiliations.
Supplementary information
Supplementary legends., supplementary figure 1., supplementary figure 2., rights and permissions.
Open Access This article is licensed under a Creative Commons Attribution 4.0 International License, which permits use, sharing, adaptation, distribution and reproduction in any medium or format, as long as you give appropriate credit to the original author(s) and the source, provide a link to the Creative Commons licence, and indicate if changes were made. The images or other third party material in this article are included in the article's Creative Commons licence, unless indicated otherwise in a credit line to the material. If material is not included in the article's Creative Commons licence and your intended use is not permitted by statutory regulation or exceeds the permitted use, you will need to obtain permission directly from the copyright holder. To view a copy of this licence, visit http://creativecommons.org/licenses/by/4.0/ .
Reprints and permissions
About this article
Cite this article.
Zyrianova, T., Lopez, B., Olcese, R. et al. K 2P 2.1 (TREK-1) potassium channel activation protects against hyperoxia-induced lung injury. Sci Rep 10 , 22011 (2020). https://doi.org/10.1038/s41598-020-78886-y
Download citation
Received : 24 June 2020
Accepted : 01 December 2020
Published : 15 December 2020
DOI : https://doi.org/10.1038/s41598-020-78886-y
Share this article
Anyone you share the following link with will be able to read this content:
Sorry, a shareable link is not currently available for this article.
Provided by the Springer Nature SharedIt content-sharing initiative
This article is cited by
Salidroside attenuates hali via il-17a-mediated ferroptosis of alveolar epithelial cells by regulating act1-traf6-p38 mapk pathway.
- Zhongfu Zuo
- Zhansheng Hu
Cell Communication and Signaling (2022)
Emerging roles of mechanosensitive ion channels in acute lung injury/acute respiratory distress syndrome
- Zhiqiang Hu
Respiratory Research (2022)
Mechanism of Adipose-Derived Mesenchymal Stem Cell-Derived Extracellular Vesicles Carrying miR-21-5p in Hyperoxia-Induced Lung Injury
- Zhihui Zhang
Stem Cell Reviews and Reports (2022)
By submitting a comment you agree to abide by our Terms and Community Guidelines . If you find something abusive or that does not comply with our terms or guidelines please flag it as inappropriate.
Quick links
- Explore articles by subject
- Guide to authors
- Editorial policies
Sign up for the Nature Briefing newsletter — what matters in science, free to your inbox daily.

REVIEW article
Role of trek-1 in health and disease, focus on the central nervous system.
- Centre National de la Recherche Scientifique, Institut de Pharmacologie Moléculaire et Cellulaire, UMR7275, Université Côte d’Azur, Valbonne, France
TREK-1 is the most studied background K 2P channel. Its main role is to control cell excitability and maintain the membrane potential below the threshold of depolarization. TREK-1 is multi-regulated by a variety of physical and chemical stimuli which makes it a very promising and challenging target in the treatment of several pathologies. It is mainly expressed in the brain but also in heart, smooth muscle cells, endocrine pancreas, and prostate. In the nervous system, TREK-1 is involved in many physiological and pathological processes such as depression, neuroprotection, pain, and anesthesia. These properties explain why many laboratories and pharmaceutical companies have been focusing their research on screening and developing highly efficient modulators of TREK-1 channels. In this review, we summarize the different roles of TREK-1 that have been investigated so far in attempt to characterize pharmacological tools and new molecules to modulate cellular functions controlled by TREK-1.
Introduction
In the brain, ion channels regulate a variety of cellular processes such as neurotransmitter release, neuronal excitability, and plasticity. They are crucial in the generation and propagation of action potentials. Dysfunction of these channels causes several pathologies called channelopathies. In order to treat these channelopathies, many ion channels have been targets of small molecules and biological drugs. Overall, ion channels represent 19% of all human protein targets ( Santos et al., 2017 ). To date, 177 ion channels-based drugs have been approved as treatments for various pathologies and disorders ( Santos et al., 2017 ). Central nervous system (CNS) pathologies were associated with dysregulation of ion channels such as voltage gated Na + , K + , and Ca 2+ channels. Calcium channels are the most studied among ion channels since Ca 2+ ions in addition to controlling the cell excitability, they act as second messengers that convert electrical signals into chemical activity ( Pietrobon, 2002 ). On the other hand, to control neuronal excitability, potassium channels repolarize neurons by extruding K + ions to the extracellular space. Neurological potassium channelopathies are mainly caused by dysregulation of voltage-dependent potassium channels ( Benatar, 2000 ) and also of the inwardly rectifying potassium channels (Kir) which provokes an hyperexcitability seen in epilepsy ( Spillane et al., 2016 ). Mutations in the KCNT1 gene encoding for sodium -activated potassium channels cause a rare infantile encephalopathy called the migrating partial seizures of infancy ( Barcia et al., 2012 ). In other cases, mutations of KCNQ2 encoding for Kv7.2 channel provoke severe epileptic encephalopathies ( Weckhuysen et al., 2012 ). The inward-rectifier potassium channel Kir 6.2 is encoded by KCNJ11 gene forms the major subunit of the ATP-sensitive potassium channel. Mutations in this gene cause DEND syndrome, a very rare severe form of neonatal diabetes mellitus characterized by developmental delay, epilepsy and neonatal diabetes ( Gloyn et al., 2004 ). Kv7.2 and Kv7.3 are the main components of the slow voltage-gated M-channel which regulates neuronal excitability ( Brown and Passmore, 2009 ). Loss of function mutations of the KCNQ2 and KCNQ3 genes encoding, respectively, for Kv7.2 and Kv7.3 causes benign familial neonatal convulsions ( Spillane et al., 2016 ). A dominant missense mutation in KCNMA1 gene encoding for pore-forming α-subunit of the large conductance calcium-sensitive potassium channel (BK), has been associated with a form of generalized epilepsy and paroxysmal dyskinesia ( Du et al., 2005 ).
Some mutations in K 2P channels were reported to cause several pathologies. Birk Barel mental retardation dysmorphism syndrome is caused by a missense mutation in the maternal copy of KCNK9 gene which encodes for TASK-3 (K 2P 9.1). Loss of function of the channel was observed in homodimers and also when it forms heterodimer with TASK-1 (K 2P .3.1) ( Barel et al., 2008 ). Recently, de novo a gain of function missense mutation of KCNK4 gene encoding for TRAAK channel, was reported to cause recognizable neurodevelopmental syndrome characterized by a facial dysmorphism, hypertrichosis, epilepsy, intellectual disability/developmental delay and gingival overgrowth ( Bauer et al., 2018 ). A frameshift mutation (F139Wfsx24) in KCNK18 encoding for the calcium-activated K 2P channel TRESK channel was associated with migraine with aura ( Lafreniere et al., 2010 ). Channelopathies caused by dysfunction of potassium channels are of high interest for researchers since they may present interesting targets for potential treatments.
The most recent discovered family of two-pore domain potassium channels (K 2P ) are regulated by a variety of chemical and physical stimuli ( Lesage and Lazdunski, 2000 ). In this review, we will focus on describing the role of one of the most studied K 2P channel, TREK-1 channel in health and disease. In addition to discussing the recent pharmacological modulators of its activity.
Trek-1 Channel
TREK-1, named KCNK2 or K 2P 2.1 belongs to a large family of K 2P channels containing 15 members grouped in six subfamilies. K 2P channels are the most recent class of K + channels discovered. K 2P channels or the two-pore domain potassium channels are tandems of four transmembrane segments (M1–M4) containing two-pore domain (P1 and P2) ( Figures 1 , 2 ). They possess an extended M1-P1 extracellular loop and cytosolic N- and C-termini. K 2P channels have a unique pore signature sequence Gly-Tyr(Phe)-Gly in the 1st pore (P1) and Gly-Leu(Phe)-Gly in the 2nd pore (P2) ( Honore, 2007 ; Figure 1 ). TREK-1 was first cloned from the mouse brain ( Fink et al., 1996 ; Figure 3 ). TREK-1 was named after TWIK-1 channel the first cloned K 2P channel ( Lesage et al., 1996 ). TREK-1 shares 28% sequence homology with TWIK-1 channel. TREK-1 is highly expressed in brain and lung, but is also present in kidney, heart and skeletal muscle. When we look at the brain localization, TREK-1 is highly expressed in several regions of the brain such as the olfactory bulb, the hippocampus, the cerebellum and the cortex ( Fink et al., 1996 ).
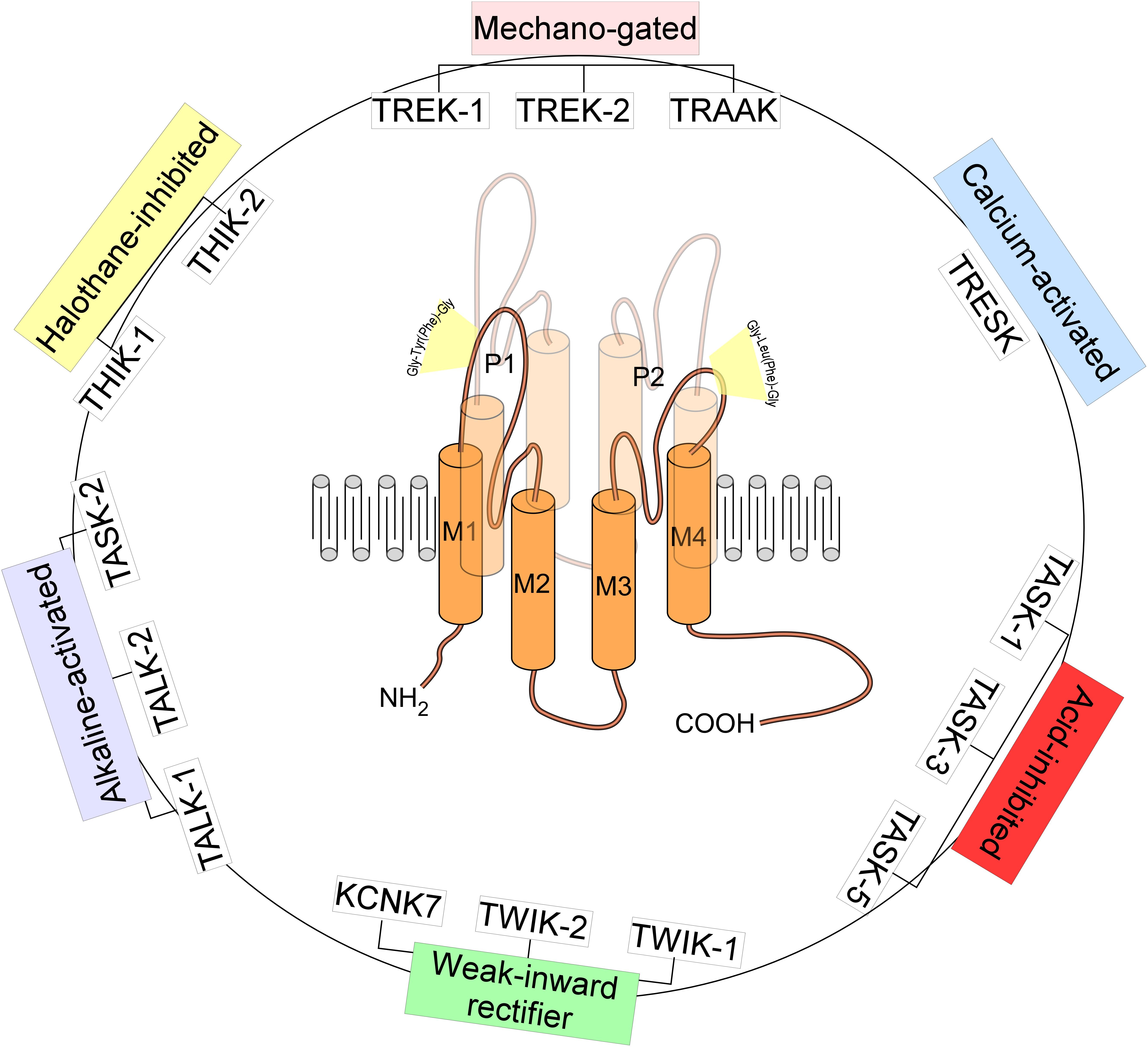
Figure 1. Structure and classification of K 2P channels. The family of K 2P channels is composed of 15 members grouped in six subfamilies. K 2P channels are two-pore domain potassium channels and the most recent class of K + channels discovered. They assemble as dimers of four transmembrane segments (M1–M4) and two-pore domain (P1 and P2). They have an extended M1-P1 extracellular loop and cytosolic N- and C-termini. K 2P channels have a unique pore signature sequence Gly-Tyr(Phe)-Gly in the 1st pore (P1) and Gly-Leu(Phe)-Gly in the 2nd pore (P2).
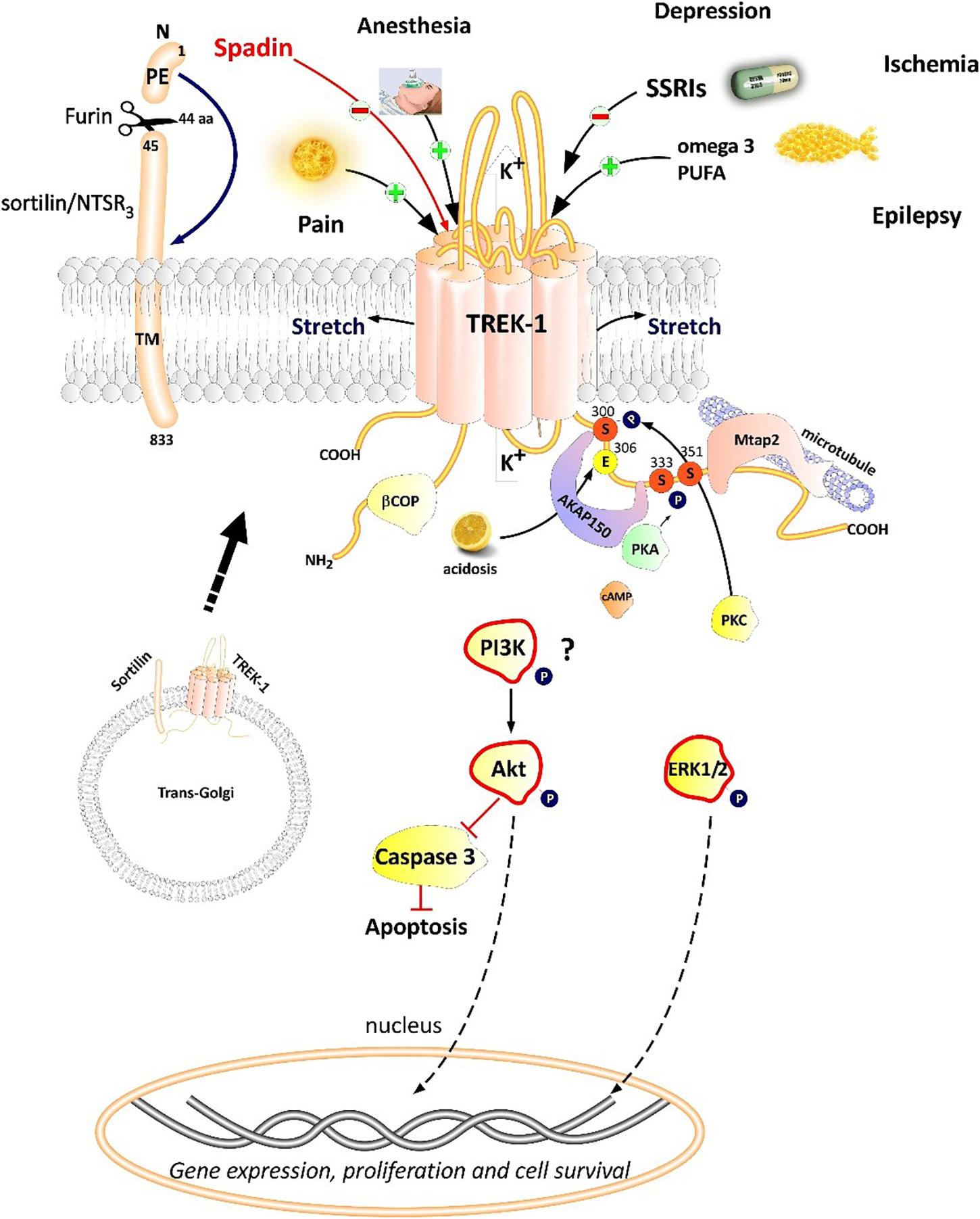
Figure 2. Polymodal TREK-1 regulation. TREK-1 is multiregulated by a variety of physical and chemical stimuli. TREK-1 possesses different protein partners such as AKAP150, β-COP, Mtap2, and sortilin. Sortilin interacts with TREK-1 an address it to the plasma membrane. Spadin is a synthetic peptide derived from sortilin which was shown to block TREK-1 with high affinity. Spadin antidepressant activity appears to be mediated through PI3K and Akt activation. TREK-1 is involved in numerous CNS pathologies such as depression, ischemia, epilepsy and pain.
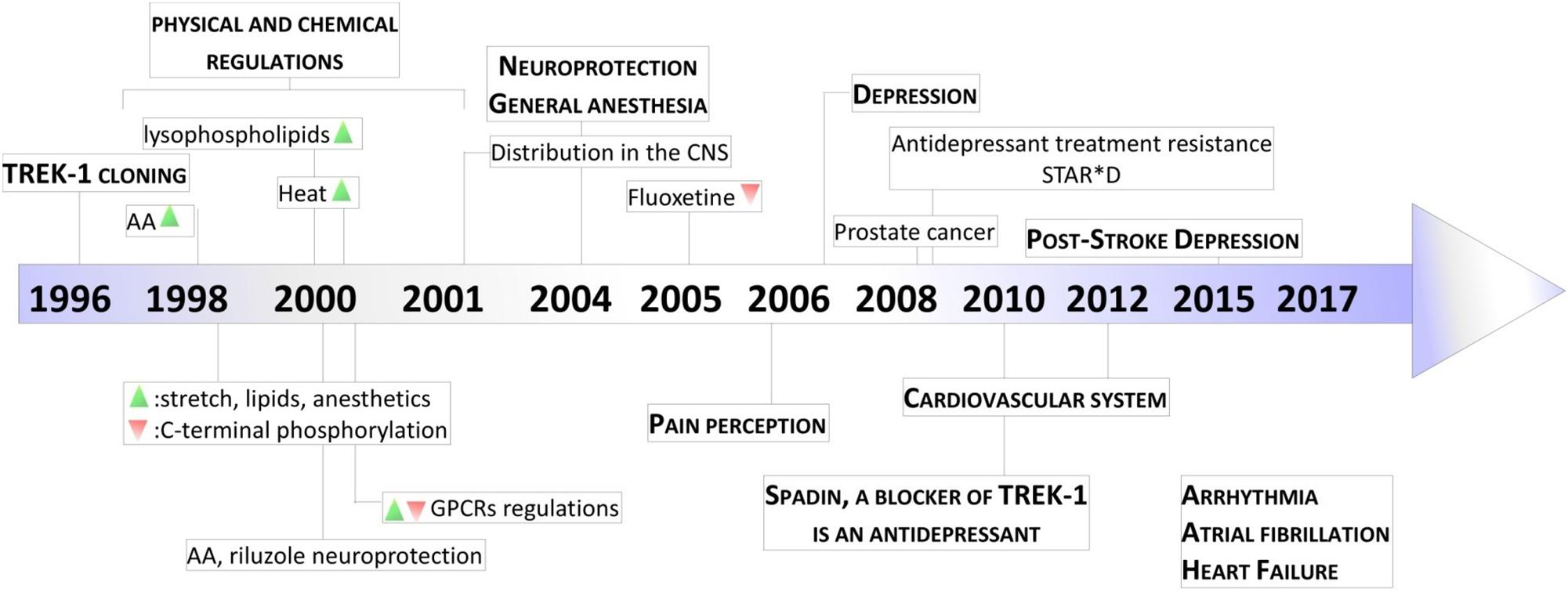
Figure 3. Key milestones in discovery of TREK-1 channels. The scheme presents the major dates from the cloning of TREK-1 channels to the discovery of its role in physiology and pathology. AA, arachidonic acid; GPCR, G-protein Coupled Receptor; STAR ∗ D, Sequenced Treatment Alternatives to Relieve Depression. Green and Red triangles represent TREK-1 activation and inhibition processes, respectively.
TREK-1 displays an outward rectification in symmetrical K + condition due to an external Mg 2+ block present at negative potential and to a voltage-dependence mechanism ( Maingret et al., 2002 ). Despite the absence of a voltage-sensing domain in K 2P channels, TREK-1 and some other K 2P channels show a strong voltage-dependency. The provenance of this voltage sensitivity comes from an ion-flux gating mechanism and the movement of three to four K + ions into the high electric field of an inactive selectivity filter ( Schewe et al., 2016 ). However, this voltage-dependency is switched off by physiological stimuli such as arachidonic acid (AA) and Phosphatidylinositol bisphosphate (PIP 2 ), which convert TREK-1 to classical leak channels ( Schewe et al., 2016 ). TREK-1 with TREK-2 and TRAAK are mechano- and thermo-sensitive K 2P channels ( Honore, 2007 ). They are opened by stretch and cell swelling. TREK-1, TREK-2, and TRAAK knock-out mice are hypersensitive to mechanical force, they show mechanical allodynia and hyperalgesia during inflammation ( Brohawn, 2015 ). It was reported that the mechanical force is transmitted to TREK-1 and TRAAK directly through the lipid bilayer ( Brohawn et al., 2014 ). These channels open rapidly in response to tension and have a low threshold and broad range of tension activation ( Brohawn, 2015 ).
Physiological Regulation of TREK-1 Channels
TREK-1 channel is regulated by various physical and chemical stimuli ( Figure 2 ). TREK-1 is opened by mechanical stretch in cell-attached and inside-out configurations in both COS transfected cells and oocytes ( Patel et al., 1998 ). TREK-1 opening occurs at all positive and negative potentials. When the cytoskeleton is disrupted with colchicine or cytochalasin D, TREK-1 activation by stretch was not altered ( Patel et al., 1998 ). TREK-1 was shown to be sensitive to temperature variation in different type of cells expressing TREK-1. Heat gradually and reversibly activates TREK-1 reaching a maximum of activation at 37°C. However, at 12°C TREK-1 basal current is suppressed ( Maingret et al., 2000 ). TREK-1 heat activation needs cell integrity and cytosolic components since TREK-1 is insensitive to heat when patch membrane is excised ( Maingret et al., 2000 ). TREK-1 is activated by polyunsaturated fatty acids (PUFA) such as AA in different patch-clamp configurations: whole-cell, cell-attached, inside-out and outside-out configurations ( Patel et al., 1998 ). AA activates TREK-1 with a dose-dependent manner and requires C-terminal part of the channel which is crucial for the activation and the inhibition following phosphorylation by the protein kinase A (PKA). PIP 2 stimulates native striatal TREK-1 current in inside-out patch configuration ( Chemin et al., 2005 ). In Xenopus oocytes expressing TREK-1, PIP 2 hydrolysis inhibits TREK-1 channel by modulating its voltage dependence ( Lopes et al., 2005 ). It is also known that internal acidosis induces an activation of TREK-1 channels with the involvement of C-terminal part ( Maingret et al., 1999 ). TREK subfamily members including TREK-1, TREK-2, and TRAAK are sensitive to phosphatidic acid (PA) which results from the hydrolysis of phosphatidylcholine by phospholipase D (PLD). It was shown that TREK-1 and TREK-2 but not TRAAK are positively regulated by PLD2 which selectively binds to their C-terminal domains ( Comoglio et al., 2014 ).
TREK-1 Partner Proteins
Proteomic approach based on immunoprecipitation and mass spectrometry analysis of native channel complexes allowed the identification of a scaffolding protein called AKAP150 ( Sandoz et al., 2006 ; Figure 2 ). A-kinase anchoring protein (AKAP) anchors the regulatory subunit of PKA in proximity of substrates. AKAP150 forms signaling complexes within the neuron, these complexes are composed of PKA, PKC, PP2B (protein phosphatase 2B), PSD-95, SAP97 and ion channels ( Esseltine and Scott, 2013 ). When anchored by AKAP150 within the post-M4 region, TREK-1 becomes an active leak channel insensitive to the stimuli, internal acidification AA, or mechanical stretch ( Sandoz et al., 2006 ). The microtubule associated protein Mtap2 is another protein reported to associate with TREK-1 in the brain ( Sandoz et al., 2008 ). Mtap2 binds to microtubules and stabilizes them. TREK-1 and Mtap2 colocalize in several brain regions such as the hippocampus, cerebellum, olfactory bulb, striatum and cortex. Similarly to AKAP150, Mtap2 binding to TREK-1 increases its activity ( Sandoz et al., 2008 ). This increase in TREK-1 current amplitude is not due to a direct interaction with Mtap2 but results from an increase in the expression level of the channel at the plasma membrane. Within the neuron, TREK-1, AKAP150 and Mtap2 are simultaneously found in the post-synaptic terminals. This signaling complex regulates TREK-1 channel activity and its trafficking to the plasma membrane.
Using the yeast two-hybrid technique of the human cDNA library on the N-terminal region of TREK-1, a direct β-COP-TREK-1 interaction was reported ( Kim et al., 2010 ). β-COP is a subunit of Coat Protein Complex I (COPI) whose role is to form coated vesicles and manage the retrograde traffic from the Golgi back to the endoplasmic reticulum (ER) or between different compartments inside the Golgi ( Gomez-Navarro and Miller, 2016 ). β-COP depletion was found to decrease membrane expression of the cystic fibrosis transmembrane conductance regulator (CFTR) channel. Mutations in this chloride channel cause cystic fibrosis lung disease ( Rennolds et al., 2008 ). β-COP increases TREK-1 surface expression and current amplitude. β-COP is involved in the forward transport of TREK-1 through a direct interaction with N-terminal part of the channel ( Kim et al., 2010 ).
The most recently discovered protein partner of TREK-1 is sortilin ( Petersen et al., 1997 ; Figure 2 ), also known as neurotensin receptor-3 (NTSR 3 ) ( Mazella et al., 1998 ). Sortilin is synthetized as a precursor called prosortilin which is cleaved in the trans-Golgi compartment by the proprotein convertase furin to generate a mature sortilin and the release of a 44 amino-acid named the propeptide (PE) ( Munck Petersen et al., 1999 ). NTSR 3 is made of a large luminal domain, a single transmembrane segment and a short C-terminal moiety. The amount of NTSR 3 expressed at the plasma membrane does not exceed 10% while the majority is expressed intracellularly and involved mainly in intracellular trafficking. Both NTSR 3 and TREK-1 are markedly expressed in the prefrontal (PFC) and cingulate cortex, the amygdala, the hippocampus, the nucleus accumbens, the dorsal raphe nucleus (DRN) and the hypothalamus. The expression of TREK-1 was enhanced at the plasma membrane in COS-7 cells co-transfected with NTSR 3 ( Mazella et al., 2010 ).
TREK-1 Heterodimerization
The first K 2P member cloned TWIK-1 ( Lesage et al., 1996 ), is expressed with TREK-1 in astrocytes ( Zhou et al., 2009 ). The expression of a functional TWIK-1 channel necessitates its heterodimerization with TREK-1 to mediate the passive conductance in astrocytes ( Hwang et al., 2014 ). As cited earlier in this review, TREK subfamily includes TREK-1, TREK-2 and TRAAK. Despite 78% of homology between TREK-1 and TREK-2, these two lipid- and mechano-activated K + channels are differently regulated. Recent studies reported that functional TREK-1 heterodimers are formed with TREK-2 and TRAAK channels ( Blin et al., 2016 ; Levitz et al., 2016 ). The heterodimer TREK-1-TREK-2 had minimal activity at physiological pH. However, TREK-1-TREK-2 complex is activated in both alkaline and acidic environments. TREK-1-TRAAK heterodimer shows activation by both intracellular acidification and alkalinization ( Levitz et al., 2016 ). Functional TREK-1-TRAAK heterodimers are formed and present unique biophysical properties and different type of regulations ( Blin et al., 2016 ). Heteromerization of TREK subfamily leads to diversification of K 2P channels and provides different kinds of regulations. Recently, it has been shown that a truncated form (TRESK-MT) of TRESK channel, a K 2P frequently associated with migraine, heteromerizes with and inhibits TREK-1 and TREK-2 ( Royal et al., 2019 ). The heterodimer TRESK-TREK acts as a dominant negative increasing trigeminal sensory neuron excitability and migraine-like phenotype in mice ( Royal et al., 2019 ).
Pharmacology of TREK-1 Channels
Trek-1 blockers.
Since the discovery of the involvement of TREK-1 in several CNS pathologies, very high interest has been devoted to find molecules modulators of its activity. As depicted in Table 1 , search for blockers of TREK-1 activity started with the discovery of the role of TREK-1 in depression processes. Mice model of depression lacking kcnk2 (the gene encoding for TREK-1) displays a phenotype resistant to the development of depression ( Heurteaux et al., 2006 ). Spadin was the first molecule that was developed in the aim to block TREK-1 channel and mimic the antidepressant-like phenotype of kcnk2 -/- mice ( Mazella et al., 2010 ; Djillani et al., 2018 ). Spadin is a peptide synthetized from the endogenous PE released after the cleavage of prosortilin by furin. Several analogs of spadin were developed afterward to improve spadin in vivo stability and antidepressant activity ( Veyssiere et al., 2015 ; Djillani et al., 2017 ). From a library of 22 peptides, the shorter peptide sequence PE 22–28 exhibited higher inhibition potency for TREK-1 associated with an improved stability in vivo and antidepressant properties ( Djillani et al., 2017 ). Similar properties were found in its biotinylated product or analog G/A-PE 22–28 (where Gly is replaced by an Ala). These spadin analogs show an extremely high affinity for TREK-1 as inhibition occurs at nanomolar concentrations. More interesting, spadin and analogs are specific blockers for TREK-1 as no effect was observed on both TREK-2 and TRAAK, the two closest K 2P members to TREK-1 which belong to the same subfamily and share almost 80% of homology ( Moha Ou Maati et al., 2012 ; Djillani et al., 2017 ).
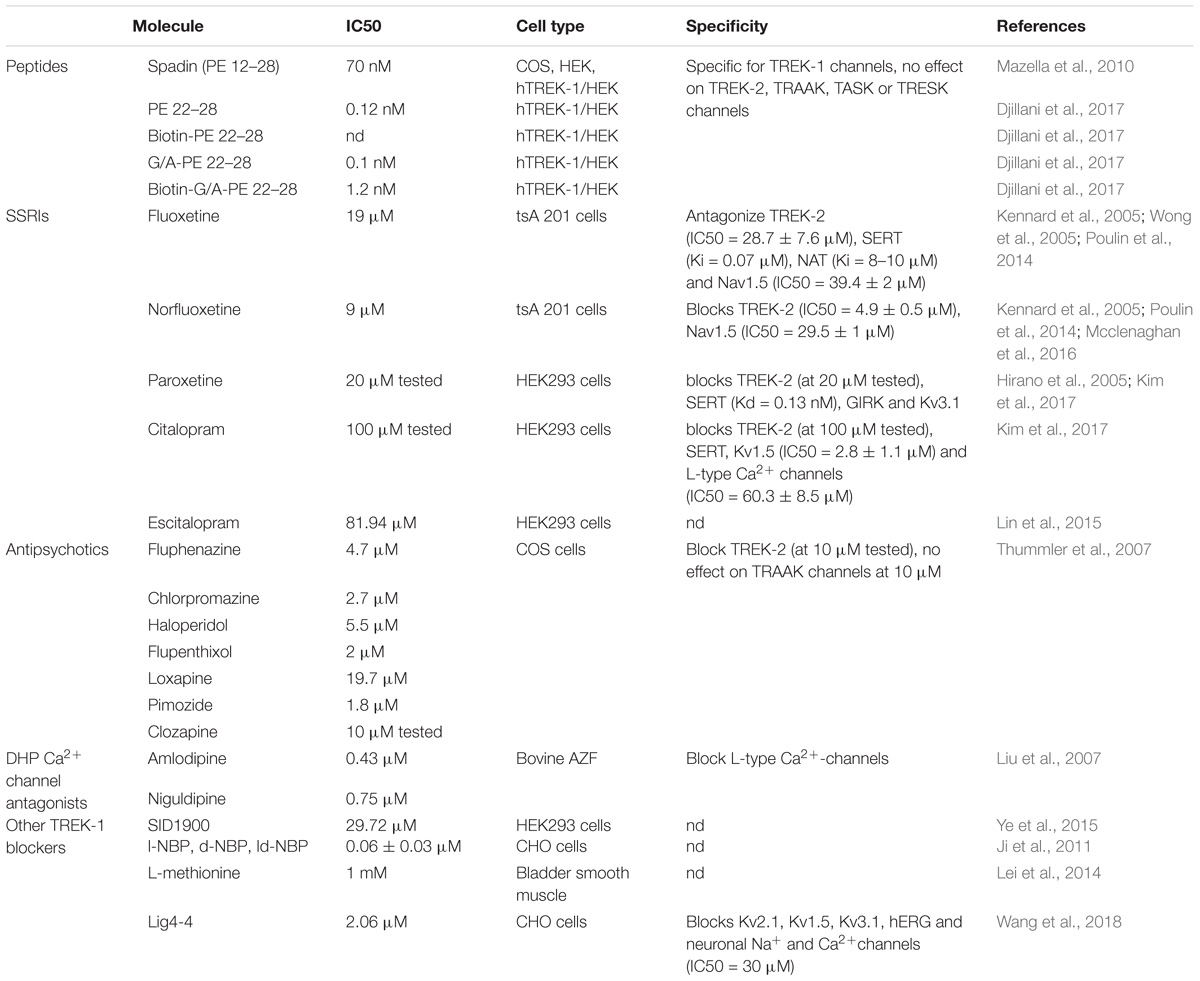
Table 1. TREK-1 blockers.
Selective Serotonin Reuptake Inhibitors (SSRI) is a family of small molecules with a high affinity for the serotonin transporter, SERT ( Hirano et al., 2005 ). SERT blockade generates an accumulation of serotonin in the synaptic clefts and displays antidepressant effects in clinic. In addition, at clinical concentrations, SSRI and fluoxetine antagonize TREK-1 channel (IC 50 = 19 μM for fluoxetine and IC 50 = 9 μM for Norfluoxetine, its active metabolite) ( Kennard et al., 2005 ). Other SSRIs such as paroxetine, citalopram or escitalopram also block TREK-1 with different potencies. However, SSRIs are not specific for TREK-1 since they target TREK-2, Nav1.5, and L-type Ca 2+ channels ( Wong et al., 2005 ; Poulin et al., 2014 ; Mcclenaghan et al., 2016 ).
Antipsychotic drugs used to treat psychosis, like schizophrenia and bipolar disorder, were shown to be potent blockers of TREK-1 channels. They antagonize TREK-1 and TREK-2 but do not affect TRAAK channels ( Table 1 ; Thummler et al., 2007 ). TREK-1 blockade was observed with both typical and atypical antipsychotic. For example, chlorpromazine and Loxapine block dose-dependently TREK-1 channels with IC 50 of 2.7 and 19.7 μM ( Thummler et al., 2007 ). These data demonstrate a potential link between TREK-1, schizophrenia and bipolar disorder.
Dihydropyridine analogs such as amlodipine or niguldipine are L-type Ca 2+ channel blockers. It has been shown that they also block TREK-1 with high affinity (IC 50 = 0.43 and 0.75 μM for amlodipine and niguldipine, respectively). In addition, mice lacking the L-type channel Cav1.3 display an antidepressant-like phenotype ( Busquet et al., 2010 ). The compound SID1900 blocks TREK-1 with an IC 50 ∼30 μM and was shown to produce antidepressant-like properties in a rat model of chronic unpredictable mild stress (CUMS) ( Ye et al., 2015 ). SID1900 effect was comparable to spadin. Still there is no evidence about other possible targets of SID1900 and more data are needed to conclude about its specificity. Finally, other molecules were reported to block TREK-1 channels and provide neuroprotection against ischemia and stroke such as 3-n-butylphtalide (NBP) and its analog lig4-4 ( Ji et al., 2011 ; Wang et al., 2018 ). However, if information is lacking about the specificity of NBP, lig4-4 was reported to affect hERG channel, voltage-gated K + channels (K v ), neuronal Na + and Ca 2+ channels, which could limit its development as potential neuroprotective molecule.
TREK-1 Activators
TREK-1 channel is activated by volatile general anesthetics such as chloroform, diethyl ether, halothane and isoflurane and it was shown that the C-terminal part is critical for its activation ( Table 2 ; Patel et al., 1999 ). If the chloroform seems to be selective for TREK-1 channels with an EC 50 (0.2–1.6 mM), halothane and isoflurane activate both TREK-1 and TASK channels. Furthermore, diethyl ether opens TREK-1 but decrease TASK channel activity ( Patel et al., 1999 ). In contrast to general anesthetics, local anesthetics like bupivacaine behave as inhibitors of TREK-1 ( Kindler et al., 1999 ). AA and other PUFAs open TREK-1 channels in dose-dependent manner ( Patel et al., 1998 ). Channel opening by AA, α-linolenic acid (ALA), and docosahexaenoic acid (DHA) is thought to contribute to neuroprotection ( Lauritzen et al., 2000 ). If SSRI antidepressants and antipsychotics drugs act as TREK-1 blockers, mood stabilizers such as lithium chloride and antiepileptics drugs like gabapentine, valproate and carbamazepine were reported to activate TREK-1 channel ( Kim et al., 2017 ). The tetrazole based compound BL-1249 showed an interesting TREK-1 activation with an EC 50 of around 1.5 μM in vitro in cultured human urinary bladder myocytes ( Tertyshnikova et al., 2005 ). In a pancreatic carcinoma cell line, BL-1249 activates dose-dependently TREK-1 with an IC 50 = 2 ± 2 μM ( Sauter et al., 2016 ). Recent studies provided more insights into the action mechanism of BL-1249. Indeed, it was shown that BL-1249 activates the selectivity filter C-type gate and activates selectively TREK-1 and TREK-2 channels 10-fold stronger than TRAAK channels ( Pope et al., 2018 ). In addition, BL-1249 action requires the C-terminal tail of the channel for the activation and the transmembrane domains M2 and M3 are critical for its selectivity ( Pope et al., 2018 ). Following the screening of more than 106,000 small molecules, an activator of TREK-1 named ML67 and its optimized analog ML67-33 were identified ( Bagriantsev et al., 2013 ). Then after, two molecules with higher potency were recently developed by the same laboratory, ML335 and ML402 which potentiate TREK-1 activity with EC 50 of 5.2 ± 0.8 μM and 5.9 ± 1.6 μM, respectively ( Table 2 ; Lolicato et al., 2017 ). ML335 and ML402 bind and activate a cryptic binding pocket within the C-type gate selectivity filter of TREK-1 channel ( Lolicato et al., 2017 ). Non-steroid anti-inflammatory drugs (NSAIDs) such as flufenamic acid, niflumic acid and mefenamic acid were reported to activate TREK-1 channels independently of cyclooxygenase COX inhibition ( Veale et al., 2014 ). Caffeic acid derivatives were reported to activates TREK-1 channel and provide potent analgesic effect in vivo ( Rodrigues et al., 2014 ; Vivier et al., 2017 ). Finally, small molecule GI-530159 was described as TREK-1 opener which reduces small DRG neurons excitability ( Loucif et al., 2018 ). However, GI-530159 is not selective for TREK-1 channels since it activates also TREK-2 with no significant effect on TRAAK channels ( Loucif et al., 2018 ).
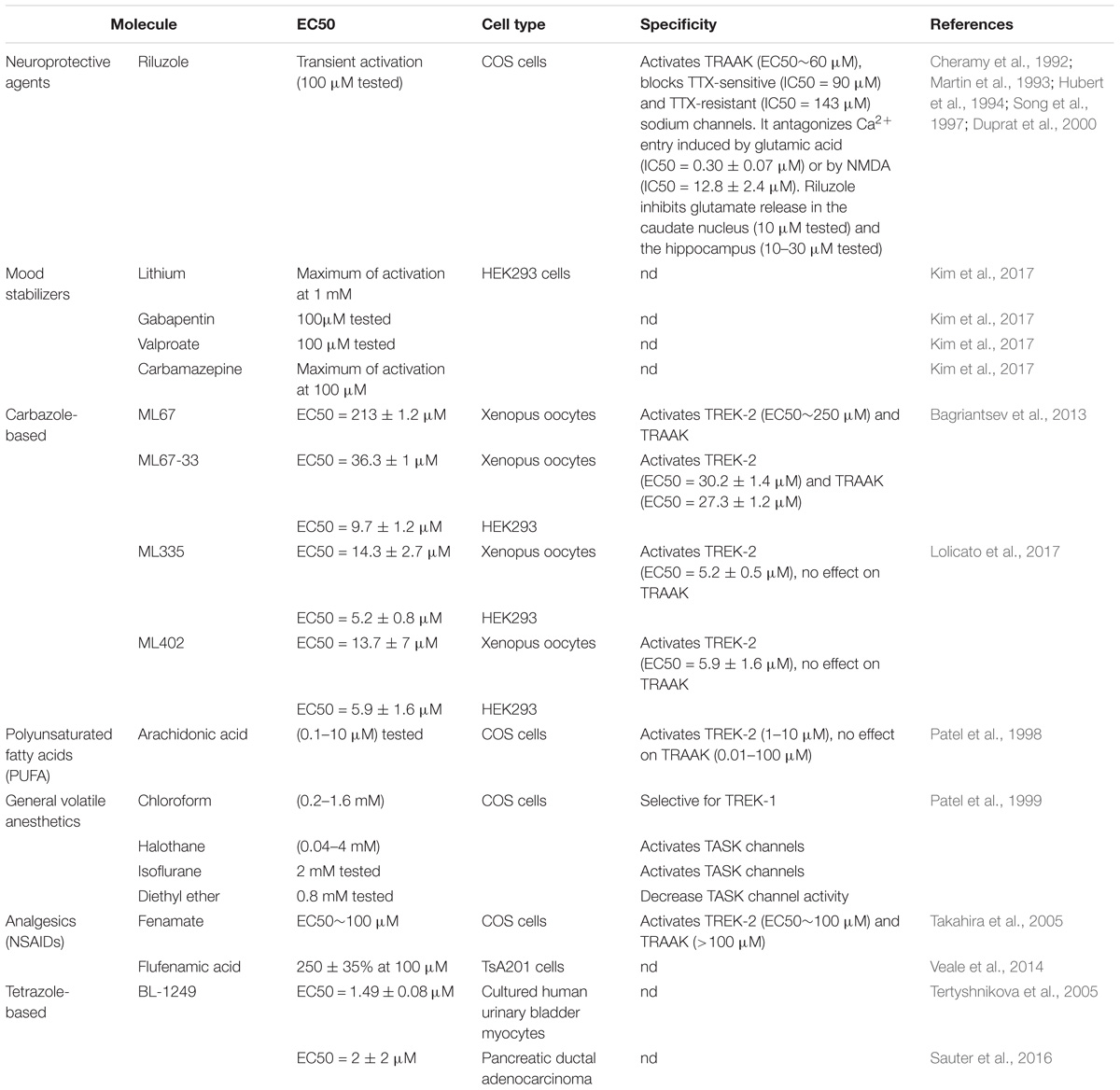
Table 2. TREK-1 activators.
TREK-1 Modulators
Riluzole ( Table 2 ) is a neuroprotective molecule marketed as an anticonvulsant drug. Its action mechanism involves the blockade of glutamate receptors. It is also prescribed to prolong the survival of patient suffering from amyotrophic lateral sclerosis. Besides, riluzole was shown to act as a transient TREK-1 activator within 30 s and strong inhibitor after 90 s ( Duprat et al., 2000 ). The dual activity on TREK-1 is thought to be mediated through cyclic Adenosine MonoPhosphate (cAMP) activation of PKA. On the other hand, riluzole produces a sustained activation of TRAAK without an inhibition.
TREK-1 and CNS Disorders
TREK-1 channels show a widespread distribution in rat and mouse brains ( Hervieu et al., 2001 ; Talley et al., 2001 ). It is rational to imagine multiple roles that TREK-1 can play in the CNS. In this part of the review, we will explain in depth why TREK-1 channel became a very promising target in numbers of pathologies that affect the CNS ( Figure 3 ).
TREK-1 in Depression
The role of TREK-1 in depression was demonstrated in mice invalidated for kcnk2 , the gene encoding for TREK-1 ( Heurteaux et al., 2006 ). TREK-1 knock-out mice displayed a phenotype resistant to depression in five different mouse models of depression: the Porsolt forced swim test (FST), the tail suspension test (TST), the conditioned suppression of motility test (CSMT), the learned helplessness test (LH), and the novelty-suppressed feeding test (NSF) ( Table 3 ; Heurteaux et al., 2006 ). The behavior of kcnk2 -/- mice was similar to those treated acutely or chronically with classical antidepressants like fluoxetine ( Heurteaux et al., 2006 ). Kcnk2 -deficient mice show an increase in serotonin (5-HT) neurotransmission in the DRN neurons. Deletion of TREK-1 increases neurogenesis induced with a chronic treatment with antidepressants ( Heurteaux et al., 2006 ). Blocking TREK-1 has become a novel strategy to design new generation of antidepressants. At the concentrations used clinically, SSRIs inhibits TREK-1 channels ( Kennard et al., 2005 ).
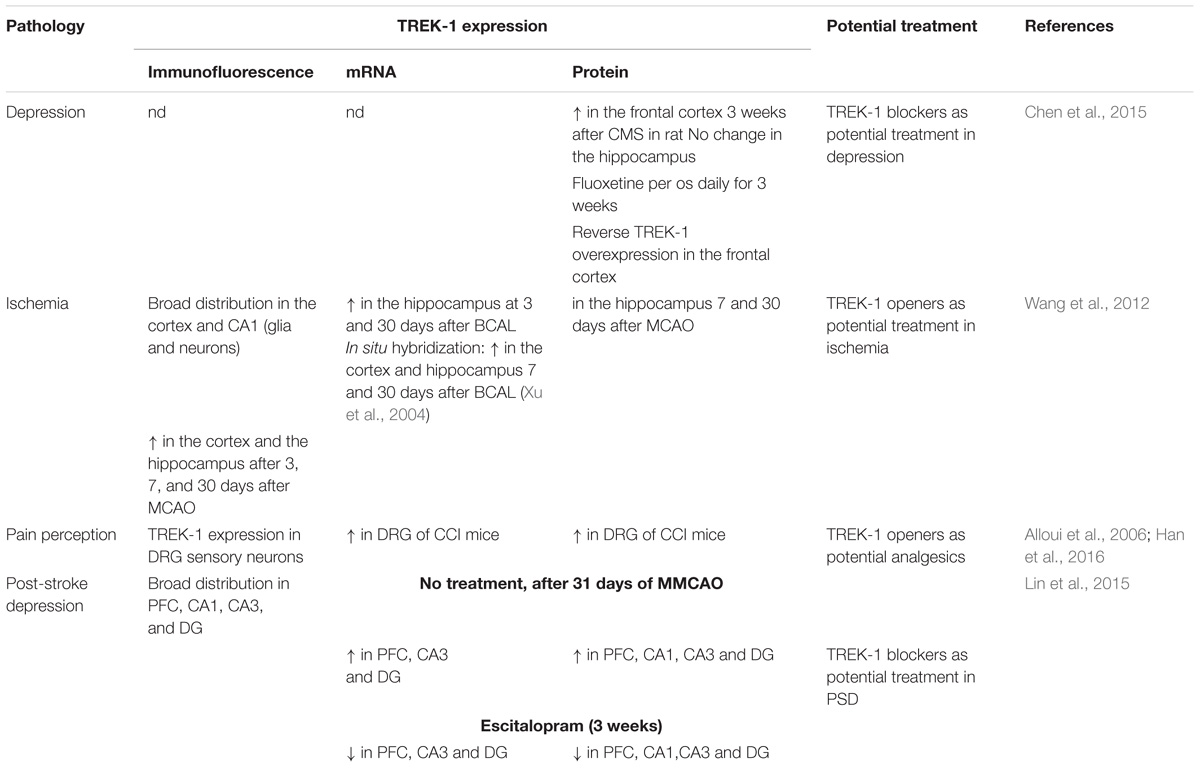
Table 3. TREK-1 in CNS pathologies.
Spadin was previously described as a specific blocker of TREK-1 with a high affinity (IC 50 = 40–70 nM) ( Mazella et al., 2010 ; Djillani et al., 2017 , 2018 ). Spadin consists in 17-amino acid peptide synthesized from the propeptide (PE). PE is 44 amino acids generated from the maturation of sortilin by the cleavage of the prosortilin with furin ( Munck Petersen et al., 1999 ). Spadin binds with high affinities both TREK-1 and sortilin/neurotensin receptor 3 (NTSR3). Spadin by blocking TREK-1 generates mice with depression-resistance phenotype in several depression tests after only 4 days of treatment. Similarly to kcnk2 -/- mice, spadin enhances 5-HT neurotransmission in DRN serotonergic neurons ( Mazella et al., 2010 ). In in vitro experiments, spadin stimulates MAPK and PI3K pathways in time and concentration-dependent manner. Spadin at 100 nM increases the phosphorylation of ERK1/2 and Akt but does not affect the phosphorylation of mTOR, suggesting an original mechanism of action of spadin different from the other fast-acting antidepressant ketamine which was demonstrated to be mTOR-dependent ( Devader et al., 2015 ). Spadin has protective effects on neurons against staurosporine-induced Caspase-3 apoptosis through the specific activation of the PI3K pathway. Spadin increases transiently and after 8 h the mRNA expression of PSD-95 and synapsin, two markers of synaptogenesis in the brain. This increase is associated with a transient BDNF increase only after 5 h. Spadin increases also the proportion of mature spines in cortical neuronal culture. In vivo , daily spadin administration for 4 days increased mRNA expression of BDNF, PSD-95 and synapsin after only 7 days in the hippocampus. However, in the prefrontal cortex (PFC), only BDNF was enhanced after 3 weeks ( Devader et al., 2015 ).
The FST antidepressant activities of spadin disappear after 7 h after an acute treatment. To improve the bioavailability and the in vivo stability of spadin, retro-inverso analogs of spadin were designed and screened on the cell line stably expressing the human TREK-1 ( Veyssiere et al., 2015 ). Two analogs, 3 and 8, were identified. They prolong the antidepressant activity from 7 to 16 h in FST. However, due to some issues concerning in vitro toxicity of analog 3 and 8 at higher concentrations, other strategies were conducted in order to ameliorate the benefit/risk ratio. By shortening spadin sequence, a peptide PE 22-28 containing only 7 amino acids was identified as the shortest efficient molecule displaying an antidepressant activity ( Djillani et al., 2017 , 2018 ). The role of TREK-1 in mice was supported by other studies in human like STAR( ∗ )D which highlights a strong association between resistance to SSRIs and the existence of four single nucleotide polymorphisms (SNP) in kcnk2 gene ( Perlis et al., 2008 ). Another SNP, rs6686529 located in kcnk2 gene was found to be associated with major depression disorder and response to antidepressant treatment ( Liou et al., 2009 ). All these data highlight the key role of TREK-1 in depression and the need to design blockers of TREK-1 as promising original antidepressant ( Figure 3 ).
TREK-1 in Neuroprotection
Trek-1 in epileptogenesis.
TREK-1 is expressed in GABA-containing interneurons in the cortex and the hippocampus ( Hervieu et al., 2001 ). In vivo , It was reported that TREK-1 activators PUFA such as α-linolenic acid protect rats treated with the glutamate receptor agonist, kainic acid (KA) against seizures and hippocampal lesions ( Lauritzen et al., 2000 ). Moreover, PUFA neuroprotection was observed in another model of seizures using glutamatergic neurons. This effect was associated with the inhibition of the glutamatergic neurotransmission ( Lauritzen et al., 2000 ). TREK-1 deficient mice were shown to be more vulnerable to develop epileptic seizures triggered by KA and pentylenetetrazol (PTZ, GABA A receptor antagonist) ( Heurteaux et al., 2004 ). The expression of c-fos , a marker of neuronal excitability was increased in CA3 pyramidal neurons following treatment with KA ( Heurteaux et al., 2004 ). Linolenic acid and lysophosphatidylcholine (LPC) decrease KA-induced seizures in wild-type mice. However, no effect was observed in mice lacking TREK-1 channels ( Heurteaux et al., 2004 ). The protective effects of linolenic acid and LPC require the presence of TREK-1 channels. Status epilepticus (SE) is a persistent repeated seizure activity for more than 5 min. Development of TREK-1 mutant (TREK-M) produced a constitutively open TREK-1 channels that showed resistance to PKA and PKC downregulation. TREK-M was able to hyperpolarize the plasma membrane and decrease spontaneous firing of hippocampal neurons in culture ( Dey et al., 2014 ). In vivo administration of a recombinant adenoassociated virus (AAV)-mediated delivery of TREK-M in both entorhinal cortex and hippocampal CA3 regions, reduced by 50% the duration of SE in a mouse model of SE induced with lithium and pilocarpine. AAV-TREK-M prevented neuronal death in both entorhinal cortex and CA3 after injection ( Table 3 ; Dey et al., 2014 ). Since TREK-1 opening results in neuroprotection against epileptic episodes, blocking this AA-activated K 2P channel could in contrast be deleterious. Surprisingly, TREK-1 antagonist spadin does not enhance seizures induced by KA or PTZ when injected to mice. More interestingly, mice treated with spadin show more resistance to develop generalized convulsions and to induce death ( Moha Ou Maati et al., 2012 ; Djillani et al., 2018 ).
TREK-1 in ischemia
Using a model of global ischemia, results from transient bilateral occlusion of common carotid arteries in wild-type and knock-out TREK-1 mice showed that 74% of TREK -/- mice died 3 days after ischemia whereas only 34% died in wild-type group ( Heurteaux et al., 2004 ). Here again, linolenic acid or LPC pretreatment were unable to protect against ischemia in TREK-1 deficient mice in contrast to wild-type mice in which mice survival was significantly increased ( Heurteaux et al., 2004 ). Spinal cord ischemia is induced in mice by occluding both the aortic arch and left sub-clavian artery. It was reported that 75% of kcnk2 -/- mice died 3 h after 10 min ischemia compared to only 14% of wild-type mice 24 h after ischemia ( Heurteaux et al., 2004 ). Furthermore, surviving TREK-1 -/- mice developed severe hind limb paralysis. However, no neurological deficit was observed with wild-type mice ( Heurteaux et al., 2004 ). In addition to neurons, astrocytes were shown to play an important role in brain ischemia. TREK-1 contributes in maintaining the highly negative membrane potential in astrocytes which is crucial in controlling cell excitability ( Zhou et al., 2009 ). In cultured cortical astrocytes and in hippocampal slices, TREK-1 is widely expressed. Cortical and hippocampal TREK-1 channels were upregulated during astrogliosis following focal ischemia ( Wang et al., 2012 ). In hypoxic conditions, TREK-1 protein expression in astrocytes was also upregulated which increases glutamate clearance, suppressed the astrocytic marker S100β and block neuronal death ( Table 3 ; Wu et al., 2013 ). Glutamate is the main excitatory neurotransmitter in the brain. Astrocytes release glutamate following GPCR activation. DH Woo et al. demonstrate that TREK-1 channel is involved in the fast glutamate release which requires the interaction between the N-terminal domain of TREK-1 and the G βγ of the G αi PCR ( Woo et al., 2012 ).
TREK-1 in general anesthesia
In general anesthesia, at clinical doses used for isoflurane, diethyl ether, halothane and chloroform, TREK-1 channels are activated ( Patel et al., 1999 ). TREK-1 channel activation implies the C-terminal moiety and leads to cell hyperpolarization, decreased action potential firing and neuroprotection. The laughing gas nitrous oxide and xenon mostly exert their anesthetic effects via antagonizing NMDA receptors since they provide analgesia, euphoria and neuroprotection. They do not potentiate GABA A receptors ( Gruss et al., 2004 ). Nitrous oxide and xenon were shown to open TREK-1 channels with Glu306 as a critical amino acid for the anesthetic activation of TREK-1 ( Gruss et al., 2004 ). Chloral hydrate is used in pediatrics in certain forms of epilepsy such as progressive myoclonus and refractory childhood epilepsies. Upon administration, Chloral hydrate is rapidly metabolized into its active metabolite 2,2,2-trichlorethanol (TCE) which is thought to be responsible for the chloral hydrate activity ( Harinath and Sikdar, 2004 ). TCE induces depression of the CNS by potentiating GABA A receptors and inhibiting NMDA, AMPA, and Kainate receptors. It was reported that TCE also activates TREK-1 and TRAAK channels and contributes to the central anesthetic effect ( Harinath and Sikdar, 2004 ). In vivo , mice lacking TREK-1 channels displayed a lower sensitivity to the most potent TREK-1 activators, chloroform and halothane and also to sevoflurane, desflurane and isoflurane ( Heurteaux et al., 2004 ). The threshold to provoke anesthesia and the doses required for the anesthetic action were higher. Phenobarbital, a general anesthetic that does not modulate TREK-1 channels, fails to reproduce these effects in kcnk2 -/- mice. Thus, the observed effects were due specifically to TREK channel absence. Recently, in vitro and in vivo preconditioning with sevoflurane was shown to provide neuroprotection through activation of TREK-1 channels ( Tong et al., 2014 ). Rats treated with the anesthetic sevoflurane after MCAO display neuroprotection which seems to be mediated by TREK-1 channels ( Table 3 ; Pan et al., 2017 ).
Role of TREK-1 in Post-stroke Depression
Given the neuroprotective role of TREK-1 channels in stroke and its involvement in the depression process. It makes sense to address the role of this K 2P channel in the physiopathology of post-stroke depression (PSD). Recently, several laboratories have started to investigate the potential role of TREK-1 channels in this pathology. PSD is a high prevalence neuropsychiatric disorder that takes place after stroke onset. It has been shown that TREK-1 channel expression levels were elevated in the hippocampus and the PFC in rat model of PSD ( Table 3 ; Lin et al., 2015 ). Noteworthy, TREK-1 channel up-regulation was suppressed following chronic treatment with the TREK-1 blocker and the SSRI, escitalopram. In this PSD rat model, the main actors causing PSD in human patients were reproduced. For example, the middle cerebral artery occlusion (MCAO) surgery was set up to mimic stroke and chronic mild stress (CMS) and isolation rearing to mimic stress and psychosocial conditions, respectively ( Lin et al., 2015 ).
TREK-1 in Pain Perception
TREK-1 channels are known to be modulated by heat. Those K 2P channels are gradually and reversibly opened by temperature. Noteworthy, TREK-1 channel opening is reversed by cAMP and prostaglandin E2, two sensitizers of peripheral and central thermoreceptors. TREK-1 blockade is due to the phosphorylation of Ser333 on its C-terminal end. The thermosensitive TREK-1 channel is highly expressed and localized in small dorsal root ganglion (DRG) neurons and in central hypothalamic neurons, areas that are highly involved in pain ( Maingret et al., 2000 ). Sixty percent of sensory neurons express TREK-1 and most of them are associated with substance P. More than 40% of DRG neurons that express TREK-1 channels also express the thermal nociceptor TRPV1 channels. In vivo , compared to wild type mice, mice lacking kcnk2 gene are highly sensitive to low-threshold thermal pain and mechanical stimuli ( Alloui et al., 2006 ). TREK-1 deficient mice showed an exacerbated focal inflammatory response after spinal cord injury (SCI). Furthermore, TREK-1 deficiency enhanced astrogliosis, neuronal apoptosis, demyelination and retarded motor recovery ( Fang et al., 2017 ). Nevertheless, in other reported studies, in the DRG, the microRNA miR-183 expression was decreased and TREK-1 expression was increased in neuropathic pain induced by chronic constriction of sciatic nerve (CCI) ( Table 3 ; Han et al., 2016 ; Shi et al., 2018 ). Intrathecal injection of miR-183 alleviated pain in rat model of CCI and downregulated TREK-1 expression ( Shi et al., 2018 ). Recently, it was reported that TREK-1 modulator riluzole prevents both sensory and motor deficits in neuropathic pain induced by the chemotherapy drug oxaliplatin ( Poupon et al., 2018 ). Nevertheless, conclusions drawn by this paper are not totally convincing because authors only consider riluzole as an activator and do not take into account its inhibitory effects. Finally, TREK-2 and TRAAK channels, the other members of TREK subfamily are characterized in the small sized DRG neurons of rats ( Viatchenko-Karpinski et al., 2018 ).
Peripheral Roles of TREK-1 Channels
Trek-1 in the heart.
In the heart, the stretch-activated K + channels (SAK) repolarize cell membrane and counterbalance the activity of stretch-activated Cation channels (SAC) which in contrast increase cation influx and cell depolarization. TREK-1 channel presents a serious candidate to form SAK in heart with the large conductance Ca 2+ -activated K + channel (BK Ca ) and K ATP channels ( Decher et al., 2017a ). In rat heart, all K 2P channel genes were detected in at least one heart chamber with a prevalence of TWIK-2, TASK-1 and TREK-1 expression. TREK-1 channel was highly expressed in the right ventricle ( Liu and Saint, 2004 ). In rat neonatal atrial myocytes, using single channel inside-out patch, TREK-1-like current was activated by AA and internal acidosis ( Kim and Clapham, 1989 ). This TREK-1-like current was shown to be reversibly activated by volatile anesthetics such as chloroform, halothane and isoflurane. It was also downregulated by cAMP analogs and β-adrenergic receptor agonists ( Terrenoire et al., 2001 ). SAK current shared identical biophysical properties with the recombinant TREK-1 current such as the single channel conductance, no voltage-dependency and sensitivity to volatile anesthetics. In the ventricular cardiomyocytes, two variants of TREK-1 were found, large conductance and small conductance with (132 ± 5 pS) and (41 ± 5 pS), respectively, at positive potentials ( Xian Tao et al., 2006 ). The low-conductance TREK-1 variant was opened by mechanical stretch, internal acidification and AA. The biophysical properties of the two TREK-1 channels were similar to those displayed by the recombinant TREK-1 channels expressed in HEK293 cells. Using cardiac specific TREK-1 deficient mice, it was proposed that TREK-1 plays an essential role in sinoatrial node cell excitability ( Unudurthi et al., 2016 ). Recently, a heterozygous point mutation in the selectivity filter of TREK-1 channel was identified in patients diagnosed with right ventricular outflow tract (RVOT) tachycardia ( Decher et al., 2017b ). More interestingly, TREK-M showed a hypersensitivity to stretch and permeability to sodium ions ( Decher et al., 2017b ; Figure 3 ).
Role of TREK-1 in Other Tissues
In addition to the central roles of TREK-1 channels in the brain, it was thoroughly rational to think that modulating TREK-1 channels could play a peripheral role as this K 2P channel is expressed in number of tissues such as pancreas, prostate and smooth muscle cells ( Medhurst et al., 2001 ). The pancreas and particularly the insulin secreting β-cells constitute one of the main targets of the peripheral tissues where TREK-1 and other K 2P channels play an important role in glucose homeostasis ( Kang et al., 2004 ; Mazella et al., 2010 ; Dadi et al., 2014 ; Vierra et al., 2015 ). Given the importance of TREK-1 channel as a key modulator of plasma membrane potential in addition to its regulation by various physical and chemical stimuli, its role in the glucose homeostasis has been investigated using spadin ( Hivelin et al., 2016 ). Spadin inhibits TREK-1 channels in the pancreatic β-cell line β-TC3 ( Mazella et al., 2010 ). It is known that the regulation of insulin secretion by pancreatic β-cell is fine-tuned by a variety of hormones such as glucagon like-peptide-1 (GLP-1), leptin, estrogen, melatonin and growth hormone ( Fu et al., 2013 ). It is well established that ATP-sensitive potassium channels (K ATP ) play a critical role in maintaining glucose homeostasis ( Mctaggart et al., 2010 ). K ATP channels serve as a metabolic sensor for the pancreatic β-cell. When glucose levels increase, K ATP channels close, inducing a membrane depolarization resulting in the opening of Ca 2+ channels as well as the secretion of numerous hormones and amplification of the effects of glucose and other secretagogues such as GLP-1 ( Mctaggart et al., 2010 ). TREK-1 blockade by spadin induces insulin secretion only upon glucose stimulation. However, in contrast to incretin hormones, spadin induces Ca 2+ increase and insulin release through an original PKA-independent mechanism ( Hivelin et al., 2016 ). TREK-1 channels are inhibited as well by the PKA phosphorylation of the Ser333 at the C-terminal moiety of the channel ( Murbartián et al., 2005 ), which is the consequence of the activation of different G-protein-coupled receptors at the plasma membrane. By binding to their GLP-1 receptors, GLP-1 and its analogs such as exendin-4 are able to trigger cAMP synthesis and thus induce PKA activation leading to insulin secretion ( Göke et al., 1993 ). Then, insulin release by β-cells is potentiated by TREK-1 closing following PKA phosphorylation. Spadin or its analogs could substitute to incretin hormone actions and could propose a therapeutic alternative in case of inefficacy of antidiabetic treatment using GLP-1 mimetics. Among the other K 2P channels, the mechano-sensitive K 2P channel TREK-1 is predominately expressed in the human detrusor channel ( Andersson and Arner, 2004 ). Recently, a study assessing the relationship between six SNP and the occurrence of overactive lower urinary tract symptoms (LUTS), revealed a strong association between TREK-1 polymorphisms (rs758937019-CT genotype and rs758937019-T allele) and overactive LUTS in human ( Nedumaran et al., 2019 ).
Conclusion and Perspectives
Since their cloning 20 years ago, the physiological importance of TREK-1 channels has continued to grow ( Figure 3 ). Today, TREK-1 channels have been shown to be important and their presence is essential in a number of physiopathological processes. Their involvement in these different processes demonstrate the necessity to design pharmacological modulators, activators or inhibitors, of these channels to correct any TREK-1-related dysfunctions.
Despites a number of studies and many molecule screenings, only few putative new drugs were identified. The activators belonging to the ML and BL series show interesting results. However, they display lower affinities (in the micromolar range) and they are not specific for TREK-1 channels as they open other K 2P channels. TREK-1 openers are needed in pathologies such as ischemia, epilepsy and pain. The challenge is to improve their affinity, specificity and ability to cross the blood-brain barrier. The new ML and BL series of TREK- activators have not yet been tested in pathologies such epilepsy and ischemia. It would be interesting to investigate any neuroprotection effects of these molecules in rodent models of epilepsy or ischemia. In the same time, their specificity should be improved to avoid any off-target effects resulting from modulating other K 2P channels.
As inhibitors, the most promising molecules are spadin and its analogs. These peptides are very specific in the K 2P family and display very high affinities, in the picomolar range for the shorter analogs ( Djillani et al., 2017 ). Depression is a complex mood disorder where different actors are involved. TREK-1 is definitely one of the most promising ion channel that plays a key role in the neurobiology of depression. TREK-1 blockers such as spadin and short analogs display a huge therapeutic value over the classical antidepressants prescribed nowadays. They are fast-acting with highly affinity and specificity for TREK-1. More interestingly, they cross the blood-brain barrier which facilitates their further optimization and development as antidepressant drugs useful in clinic.
In the future, in addition to looking for new TREK-1 modulators to treat neuropsychiatric disorders, it is relevant to study the role of TREK-1 channels in peripheral tissues and understand their involvement in physiopathology. An emerging area where TREK-1 is displaying a growing interest is the heart. TREK-1 expression has been shown to change in cardiac hypertrophy ( Wang et al., 2013 ). Furthermore, a TREK-M was found to change channel permeability to sodium and cause ventricular tachycardia ( Decher et al., 2017b ).
To conclude, the wide distribution of TREK-1 in human body, the diverse stimuli and regulations that it could respond to, and the pleiotropic roles it could play in physiology and physiopathology, make TREK-1 a promising target to explore in depth but at the same time very challenging to design modulators that are tissue-specific.
Author Contributions
AD wrote the manuscript. MB, CH, and JM corrected it.
Conflict of Interest Statement
The authors declare that the research was conducted in the absence of any commercial or financial relationships that could be construed as a potential conflict of interest.
Alloui, A., Zimmermann, K., Mamet, J., Duprat, F., Noel, J., Chemin, J., et al. (2006). TREK-1, a K+ channel involved in polymodal pain perception. EMBO J. 25, 2368–2376. doi: 10.1038/sj.emboj.7601116
PubMed Abstract | CrossRef Full Text | Google Scholar
Andersson, K. E., and Arner, A. (2004). Urinary bladder contraction and relaxation: physiology and pathophysiology. Physiol. Rev. 84, 935–986. doi: 10.1152/physrev.00038.2003
Bagriantsev, S. N., Ang, K. H., Gallardo-Godoy, A., Clark, K. A., Arkin, M. R., Renslo, A. R., et al. (2013). A high-throughput functional screen identifies small molecule regulators of temperature- and mechano-sensitive K2P channels. ACS Chem. Biol. 8, 1841–1851. doi: 10.1021/cb400289x
Barcia, G., Fleming, M. R., Deligniere, A., Gazula, V. R., Brown, M. R., Langouet, M., et al. (2012). De novo gain-of-function KCNT1 channel mutations cause malignant migrating partial seizures of infancy. Nat. Genet. 44, 1255–1259. doi: 10.1038/ng.2441
Barel, O., Shalev, S. A., Ofir, R., Cohen, A., Zlotogora, J., Shorer, Z., et al. (2008). Maternally inherited Birk Barel mental retardation dysmorphism syndrome caused by a mutation in the genomically imprinted potassium channel KCNK9. Am. J. Hum. Genet. 83, 193–199. doi: 10.1016/j.ajhg.2008.07.010
Bauer, C. K., Calligari, P., Radio, F. C., Caputo, V., Dentici, M. L., Falah, N., et al. (2018). Mutations in KCNK4 that affect gating cause a recognizable neurodevelopmental syndrome. Am. J. Hum. Genet. 103, 621–630. doi: 10.1016/j.ajhg.2018.09.001
Benatar, M. (2000). Neurological potassium channelopathies. QJM 93, 787–797. doi: 10.1093/qjmed/93.12.787
CrossRef Full Text | Google Scholar
Blin, S., Ben Soussia, I., Kim, E. J., Brau, F., Kang, D., Lesage, F., et al. (2016). Mixing and matching TREK/TRAAK subunits generate heterodimeric K2P channels with unique properties. Proc. Natl. Acad. Sci. U.S.A. 113, 4200–4205. doi: 10.1073/pnas.1522748113
Brohawn, S. G. (2015). How ion channels sense mechanical force: insights from mechanosensitive K2P channels TRAAK, TREK1, and TREK2. Ann. N. Y. Acad. Sci. 1352, 20–32. doi: 10.1111/nyas.12874
Brohawn, S. G., Su, Z., and Mackinnon, R. (2014). Mechanosensitivity is mediated directly by the lipid membrane in TRAAK and TREK1 K+ channels. Proc. Natl. Acad. Sci. U.S.A. 111, 3614–3619. doi: 10.1073/pnas.1320768111
Brown, D. A., and Passmore, G. M. (2009). Neural KCNQ (Kv7) channels. Br. J. Pharmacol. 156, 1185–1195. doi: 10.1111/j.1476-5381.2009.00111.x
Busquet, P., Nguyen, N. K., Schmid, E., Tanimoto, N., Seeliger, M. W., Ben-Yosef, T., et al. (2010). CaV1.3 L-type Ca2+ channels modulate depression-like behaviour in mice independent of deaf phenotype. Int. J. Neuropsychopharmacol. 13, 499–513. doi: 10.1017/S1461145709990368
Chemin, J., Patel, A. J., Duprat, F., Lauritzen, I., Lazdunski, M., and Honore, E. (2005). A phospholipid sensor controls mechanogating of the K+ channel TREK-1. EMBO J. 24, 44–53. doi: 10.1038/sj.emboj.7600494
Chen, C., Wang, L., Rong, X., Wang, W., and Wang, X. (2015). Effects of fluoxetine on protein expression of potassium ion channels in the brain of chronic mild stress rats. Acta Pharm. Sin. B 5, 55–61. doi: 10.1016/j.apsb.2014.12.004
Cheramy, A., Barbeito, L., Godeheu, G., and Glowinski, J. (1992). Riluzole inhibits the release of glutamate in the caudate nucleus of the cat in vivo. Neurosci. Lett. 147, 209–212. doi: 10.1016/0304-3940(92)90597-Z
Comoglio, Y., Levitz, J., Kienzler, M. A., Lesage, F., Isacoff, E. Y., and Sandoz, G. (2014). Phospholipase D2 specifically regulates TREK potassium channels via direct interaction and local production of phosphatidic acid. Proc. Natl. Acad. Sci. U.S.A. 111, 13547–13552. doi: 10.1073/pnas.1407160111
Dadi, P. K., Vierra, N. C., and Jacobson, D. A. (2014). Pancreatic β-cell-specific ablation of TASK-1 channels augments glucose-stimulated calcium entry and insulin secretion, improving glucose tolerance. Endocrinology 155, 3757–3768. doi: 10.1210/en.2013-2051
Decher, N., Kiper, A. K., and Rinne, S. (2017a). Stretch-activated potassium currents in the heart: focus on TREK-1 and arrhythmias. Prog. Biophys. Mol. Biol. 130, 223–232. doi: 10.1016/j.pbiomolbio.2017.05.005
Decher, N., Ortiz-Bonnin, B., Friedrich, C., Schewe, M., Kiper, A. K., Rinne, S., et al. (2017b). Sodium permeable and “hypersensitive” TREK-1 channels cause ventricular tachycardia. EMBO Mol. Med. 9, 403–414. doi: 10.15252/emmm.201606690
Devader, C., Khayachi, A., Veyssiere, J., Moha Ou Maati, H., Roulot, M., Moreno, S., et al. (2015). In vitro and in vivo regulation of synaptogenesis by the novel antidepressant spadin. Br. J. Pharmacol. 172, 2604–2617. doi: 10.1111/bph.13083
Dey, D., Eckle, V. S., Vitko, I., Sullivan, K. A., Lasiecka, Z. M., Winckler, B., et al. (2014). A potassium leak channel silences hyperactive neurons and ameliorates status epilepticus. Epilepsia 55, 203–213. doi: 10.1111/epi.12472
Djillani, A., Pietri, M., Mazella, J., Heurteaux, C., and Borsotto, M. (2018). Fighting against depression with TREK-1 blockers: past and future. A focus on spadin. Pharmacol. Ther. 194, 185–198. doi: 10.1016/j.pharmthera.2018.10.003
Djillani, A., Pietri, M., Moreno, S., Heurteaux, C., Mazella, J., and Borsotto, M. (2017). Shortened spadin analogs display better TREK-1 inhibition, in vivo stability and antidepressant activity. Front. Pharmacol. 8:643. doi: 10.3389/fphar.2017.00643
Du, W., Bautista, J. F., Yang, H., Diez-Sampedro, A., You, S. A., Wang, L., et al. (2005). Calcium-sensitive potassium channelopathy in human epilepsy and paroxysmal movement disorder. Nat. Genet. 37, 733–738. doi: 10.1038/ng1585
Duprat, F., Lesage, F., Patel, A. J., Fink, M., Romey, G., and Lazdunski, M. (2000). The neuroprotective agent riluzole activates the two P domain K(+) channels TREK-1 and TRAAK. Mol. Pharmacol. 57, 906–912.
PubMed Abstract | Google Scholar
Esseltine, J. L., and Scott, J. D. (2013). AKAP signaling complexes: pointing towards the next generation of therapeutic targets? Trends Pharmacol. Sci. 34, 648–655. doi: 10.1016/j.tips.2013.10.005
Fang, Y., Huang, X., Wan, Y., Tian, H., Tian, Y., Wang, W., et al. (2017). Deficiency of TREK-1 potassium channel exacerbates secondary injury following spinal cord injury in mice. J. Neurochem. 141, 236–246. doi: 10.1111/jnc.13980
Fink, M., Duprat, F., Lesage, F., Reyes, R., Romey, G., Heurteaux, C., et al. (1996). Cloning, functional expression and brain localization of a novel unconventional outward rectifier K+ channel. EMBO J. 15, 6854–6862. doi: 10.1002/j.1460-2075.1996.tb01077.x
Fu, Z., Gilbert, E. R., and Liu, D. (2013). Regulation of insulin synthesis and secretion and pancreatic Beta-cell dysfunction in diabetes. Curr. Diabetes Rev. 9, 25–53. doi: 10.2174/1573399811309010025
Gloyn, A. L., Pearson, E. R., Antcliff, J. F., Proks, P., Bruining, G. J., Slingerland, A. S., et al. (2004). Activating mutations in the gene encoding the ATP-sensitive potassium-channel subunit Kir6.2 and permanent neonatal diabetes. N. Engl. J. Med. 350, 1838–1849. doi: 10.1056/NEJMoa032922
Göke, R., Fehmann, H. C., Linn, T., Schmidt, H., Krause, M., Eng, J., et al. (1993). Exendin-4 is a high potency agonist and truncated exendin-(9–39)-amide an antagonist at the glucagon-like peptide 1-(7–36)-amide receptor of insulin-secreting β-cells. J. Biol. Chem. 268, 19650–19655.
Gomez-Navarro, N., and Miller, E. A. (2016). COP-coated vesicles. Curr. Biol. 26, R54–R57. doi: 10.1016/j.cub.2015.12.017
Gruss, M., Bushell, T. J., Bright, D. P., Lieb, W. R., Mathie, A., and Franks, N. P. (2004). Two-pore-domain K+ channels are a novel target for the anesthetic gases xenon, nitrous oxide, and cyclopropane. Mol. Pharmacol. 65, 443–452. doi: 10.1124/mol.65.2.443
Han, H. J., Lee, S. W., Kim, G. T., Kim, E. J., Kwon, B., Kang, D., et al. (2016). Enhanced Expression of TREK-1 Is related with chronic constriction injury of neuropathic pain mouse model in dorsal root ganglion. Biomol. Ther. 24, 252–259. doi: 10.4062/biomolther.2016.038
Harinath, S., and Sikdar, S. K. (2004). Trichloroethanol enhances the activity of recombinant human TREK-1 and TRAAK channels. Neuropharmacology 46, 750–760. doi: 10.1016/j.neuropharm.2003.11.023
Hervieu, G. J., Cluderay, J. E., Gray, C. W., Green, P. J., Ranson, J. L., Randall, A. D., et al. (2001). Distribution and expression of TREK-1, a two-pore-domain potassium channel, in the adult rat CNS. Neuroscience 103, 899–919. doi: 10.1016/S0306-4522(01)00030-6
Heurteaux, C., Guy, N., Laigle, C., Blondeau, N., Duprat, F., Mazzuca, M., et al. (2004). TREK-1, a K+ channel involved in neuroprotection and general anesthesia. EMBO J. 23, 2684–2695. doi: 10.1038/sj.emboj.7600234
Heurteaux, C., Lucas, G., Guy, N., El Yacoubi, M., Thummler, S., Peng, X. D., et al. (2006). Deletion of the background potassium channel TREK-1 results in a depression-resistant phenotype. Nat. Neurosci. 9, 1134–1141. doi: 10.1038/nn1749
Hirano, K., Kimura, R., Sugimoto, Y., Yamada, J., Uchida, S., Kato, Y., et al. (2005). Relationship between brain serotonin transporter binding, plasma concentration and behavioural effect of selective serotonin reuptake inhibitors. Br. J. Pharmacol. 144, 695–702. doi: 10.1038/sj.bjp.0706108
Hivelin, C., Béraud-Dufour, S., Devader, C., Abderrahmani, A., Moreno, S., Moha Ou Maati, H., et al. (2016). Potentiation of calcium influx and insulin secretion in pancreatic beta cell by the specific TREK-1 blocker spadin. J. Diabetes Res. 2016:3142175. doi: 10.1155/2016/3142175
Honore, E. (2007). The neuronal background K2P channels: focus on TREK1. Nat. Rev. Neurosci. 8, 251–261. doi: 10.1038/nrn2117
Hubert, J. P., Delumeau, J. C., Glowinski, J., Premont, J., and Doble, A. (1994). Antagonism by riluzole of entry of calcium evoked by NMDA and veratridine in rat cultured granule cells: evidence for a dual mechanism of action. Br. J. Pharmacol. 113, 261–267. doi: 10.1111/j.1476-5381.1994.tb16203.x
Hwang, E. M., Kim, E., Yarishkin, O., Woo, D. H., Han, K. S., Park, N., et al. (2014). A disulphide-linked heterodimer of TWIK-1 and TREK-1 mediates passive conductance in astrocytes. Nat. Commun. 5:3227. doi: 10.1038/ncomms4227
Ji, X. C., Zhao, W. H., Cao, D. X., Shi, Q. Q., and Wang, X. L. (2011). Novel neuroprotectant chiral 3-n-butylphthalide inhibits tandem-pore-domain potassium channel TREK-1. Acta Pharmacol. Sin. 32, 182–187. doi: 10.1038/aps.2010.210
Kang, D., Choe, C., and Kim, D. (2004). Functional expression of TREK-2 in insulin-secreting MIN6 cells. Biochem. Biophys. Res. Commun. 323, 323–331. doi: 10.1016/j.bbrc.2004.08.089
Kennard, L. E., Chumbley, J. R., Ranatunga, K. M., Armstrong, S. J., Veale, E. L., and Mathie, A. (2005). Inhibition of the human two-pore domain potassium channel, TREK-1, by fluoxetine and its metabolite norfluoxetine. Br. J. Pharmacol. 144, 821–829. doi: 10.1038/sj.bjp.0706068
Kim, D., and Clapham, D. E. (1989). Potassium channels in cardiac cells activated by arachidonic acid and phospholipids. Science 244, 1174–1176. doi: 10.1126/science.2727703
Kim, E., Hwang, E. M., Yarishkin, O., Yoo, J. C., Kim, D., Park, N., et al. (2010). Enhancement of TREK1 channel surface expression by protein-protein interaction with beta-COP. Biochem. Biophys. Res. Commun. 395, 244–250. doi: 10.1016/j.bbrc.2010.03.171
Kim, E. J., Lee, D. K., Hong, S. G., Han, J., and Kang, D. (2017). Activation of TREK-1, but Not TREK-2, channel by mood stabilizers. Int. J. Mol. Sci. 18:E2460. doi: 10.3390/ijms18112460
Kindler, C. H., Yost, C. S., and Gray, A. T. (1999). Local anesthetic inhibition of baseline potassium channels with two pore domains in tandem. Anesthesiology 90, 1092–1102. doi: 10.1097/00000542-199904000-00024
Lafreniere, R. G., Cader, M. Z., Poulin, J. F., Andres-Enguix, I., Simoneau, M., Gupta, N., et al. (2010). A dominant-negative mutation in the TRESK potassium channel is linked to familial migraine with aura. Nat. Med. 16, 1157–1160. doi: 10.1038/nm.2216
Lauritzen, I., Blondeau, N., Heurteaux, C., Widmann, C., Romey, G., and Lazdunski, M. (2000). Polyunsaturated fatty acids are potent neuroprotectors. EMBO J. 19, 1784–1793. doi: 10.1093/emboj/19.8.1784
Lei, Q., Pan, X. Q., Chang, S., Malkowicz, S. B., Guzzo, T. J., and Malykhina, A. P. (2014). Response of the human detrusor to stretch is regulated by TREK-1, a two-pore-domain (K2P) mechano-gated potassium channel. J. Physiol. 592, 3013–3030. doi: 10.1113/jphysiol.2014.271718
Lesage, F., Guillemare, E., Fink, M., Duprat, F., Lazdunski, M., Romey, G., et al. (1996). TWIK-1, a ubiquitous human weakly inward rectifying K+ channel with a novel structure. EMBO J. 15, 1004–1011. doi: 10.1002/j.1460-2075.1996.tb00437.x
Lesage, F., and Lazdunski, M. (2000). Molecular and functional properties of two-pore-domain potassium channels. Am. J. Physiol. Renal Physiol. 279, F793–F801. doi: 10.1152/ajprenal.2000.279.5.F793
Levitz, J., Royal, P., Comoglio, Y., Wdziekonski, B., Schaub, S., Clemens, D. M., et al. (2016). Heterodimerization within the TREK channel subfamily produces a diverse family of highly regulated potassium channels. Proc. Natl. Acad. Sci. U.S.A. 113, 4194–4199. doi: 10.1073/pnas.1522459113
Lin, D. H., Zhang, X. R., Ye, D. Q., Xi, G. J., Hui, J. J., Liu, S. S., et al. (2015). The role of the two-pore domain potassium channel TREK-1 in the therapeutic effects of escitalopram in a rat model of poststroke depression. CNS Neurosci. Ther. 21, 504–512. doi: 10.1111/cns.12384
Liou, Y. J., Chen, T. J., Tsai, S. J., Yu, Y. W., Cheng, C. Y., and Hong, C. J. (2009). Support for the involvement of the KCNK2 gene in major depressive disorder and response to antidepressant treatment. Pharmacogenet. Genomics 19, 735–741. doi: 10.1097/FPC.0b013e32832cbe61
Liu, H., Enyeart, J. A., and Enyeart, J. J. (2007). Potent inhibition of native TREK-1 K+ channels by selected dihydropyridine Ca2+ channel antagonists. J. Pharmacol. Exp. Ther. 323, 39–48. doi: 10.1124/jpet.107.125245
Liu, W., and Saint, D. A. (2004). Heterogeneous expression of tandem-pore K+ channel genes in adult and embryonic rat heart quantified by real-time polymerase chain reaction. Clin. Exp. Pharmacol. Physiol. 31, 174–178. doi: 10.1111/j.1440-1681.2004.03964.x
Lolicato, M., Arrigoni, C., Mori, T., Sekioka, Y., Bryant, C., Clark, K. A., et al. (2017). K2P2.1 (TREK-1)-activator complexes reveal a cryptic selectivity filter binding site. Nature 547, 364–368. doi: 10.1038/nature22988
Lopes, C. M., Rohacs, T., Czirjak, G., Balla, T., Enyedi, P., and Logothetis, D. E. (2005). PIP2 hydrolysis underlies agonist-induced inhibition and regulates voltage gating of two-pore domain K+ channels. J. Physiol. 564, 117–129. doi: 10.1113/jphysiol.2004.081935
Loucif, A. J. C., Saintot, P. P., Liu, J., Antonio, B. M., Zellmer, S. G., Yoger, K., et al. (2018). GI-530159, a novel, selective, mechanosensitive two-pore-domain potassium (K2P ) channel opener, reduces rat dorsal root ganglion neuron excitability. Br. J. Pharmacol. 175, 2272–2283. doi: 10.1111/bph.14098
Maingret, F., Honore, E., Lazdunski, M., and Patel, A. J. (2002). Molecular basis of the voltage-dependent gating of TREK-1, a mechano-sensitive K(+) channel. Biochem. Biophys. Res. Commun. 292, 339–346. doi: 10.1006/bbrc.2002.6674
Maingret, F., Lauritzen, I., Patel, A. J., Heurteaux, C., Reyes, R., Lesage, F., et al. (2000). TREK-1 is a heat-activated background K(+) channel. EMBO J. 19, 2483–2491. doi: 10.1093/emboj/19.11.2483
Maingret, F., Patel, A. J., Lesage, F., Lazdunski, M., and Honore, E. (1999). Mechano- or acid stimulation, two interactive modes of activation of the TREK-1 potassium channel. J. Biol. Chem. 274, 26691–26696. doi: 10.1074/jbc.274.38.26691
Martin, D., Thompson, M. A., and Nadler, J. V. (1993). The neuroprotective agent riluzole inhibits release of glutamate and aspartate from slices of hippocampal area CA1. Eur. J. Pharmacol. 250, 473–476. doi: 10.1016/0014-2999(93)90037-I
Mazella, J., Petrault, O., Lucas, G., Deval, E., Beraud-Dufour, S., Gandin, C., et al. (2010). Spadin, a sortilin-derived peptide, targeting rodent TREK-1 channels: a new concept in the antidepressant drug design. PLoS Biol. 8:e1000355. doi: 10.1371/journal.pbio.1000355
Mazella, J., Zsürger, N., Navarro, V., Chabry, J., Kaghad, M., Caput, D., et al. (1998). The 100-kDa neurotensin receptor is gp95/sortilin, a non-G-protein-coupled receptor. J. Biol. Chem. 273, 26273–26276. doi: 10.1074/jbc.273.41.26273
Mcclenaghan, C., Schewe, M., Aryal, P., Carpenter, E. P., Baukrowitz, T., and Tucker, S. J. (2016). Polymodal activation of the TREK-2 K2P channel produces structurally distinct open states. J. Gen. Physiol. 147, 497–505. doi: 10.1085/jgp.201611601
McTaggart, J. S., Clark, R. H., and Ashcroft, F. M. (2010). The role of the K ATP channel in glucose homeostasis in health and disease: more than meets the islet. J. Physiol. 588, 3201–3209. doi: 10.1113/jphysiol.2010.191767
Medhurst, A. D., Rennie, G., Chapman, C. G., Meadows, H., Duckworth, M. D., Kelsell, R. E., et al. (2001). Distribution analysis of human two pore domain potassium channels in tissues of the central nervous system and periphery. Brain Res. Mol. Brain Res. 86, 101–114. doi: 10.1016/S0169-328X(00)00263-1
Moha Ou Maati, H., Veyssiere, J., Labbal, F., Coppola, T., Gandin, C., Widmann, C., et al. (2012). Spadin as a new antidepressant: absence of TREK-1-related side effects. Neuropharmacology 62, 278–288. doi: 10.1016/j.neuropharm.2011.07.019
Munck Petersen, C., Nielsen, M. S., Jacobsen, C., Tauris, J., Jacobsen, L., Gliemann, J., et al. (1999). Propeptide cleavage conditions sortilin/neurotensin receptor-3 for ligand binding. EMBO J. 18, 595–604. doi: 10.1093/emboj/18.3.595
Murbartián, J., Lei, Q., Sando, J. J., and Bayliss, D. A. (2005). Sequential phosphorylation mediates receptor- and kinase-induced inhibition of TREK-1 background potassium channels. J. Biol. Chem. 280, 30175–30184. doi: 10.1074/jbc.M503862200
Nedumaran, B., Pineda, R. H., Rudra, P., Lee, S., and Malykhina, A. P. (2019). Association of genetic polymorphisms in the pore domains of mechano-gated TREK-1 channel with overactive lower urinary tract symptoms in humans. Neurourol. Urodyn. 38, 144–150. doi: 10.1002/nau.23862
Pan, L., Yang, F., Lu, C., Jia, C., Wang, Q., and Zeng, K. (2017). Effects of sevoflurane on rats with ischemic brain injury and the role of the TREK-1 channel. Exp. Ther. Med. 14, 2937–2942. doi: 10.3892/etm.2017.4906
Patel, A. J., Honore, E., Lesage, F., Fink, M., Romey, G., and Lazdunski, M. (1999). Inhalational anesthetics activate two-pore-domain background K+ channels. Nat. Neurosci. 2, 422–426. doi: 10.1038/8084
Patel, A. J., Honore, E., Maingret, F., Lesage, F., Fink, M., Duprat, F., et al. (1998). A mammalian two pore domain mechano-gated S-like K+ channel. EMBO J. 17, 4283–4290. doi: 10.1093/emboj/17.15.4283
Perlis, R. H., Moorjani, P., Fagerness, J., Purcell, S., Trivedi, M. H., Fava, M., et al. (2008). Pharmacogenetic analysis of genes implicated in rodent models of antidepressant response: association of TREK1 and treatment resistance in the STAR( ∗ )D study. Neuropsychopharmacology 33, 2810–2819. doi: 10.1038/npp.2008.6
Petersen, C. M., Nielsen, M. S., Nykjær, A., Jacobsen, L., Tommerup, N., Rasmussen, H. H., et al. (1997). Molecular identification of a novel candidate sorting receptor purified from human brain by receptor-associated protein affinity chromatography. J. Biol. Chem. 272, 3599–3605. doi: 10.1074/jbc.272.6.3599
Pietrobon, D. (2002). Calcium channels and channelopathies of the central nervous system. Mol. Neurobiol. 25, 31–50. doi: 10.1385/MN:25:1:031
Pope, L., Arrigoni, C., Lou, H., Bryant, C., Gallardo-Godoy, A., Renslo, A. R., et al. (2018). Protein and Chemical Determinants of BL-1249 Action and Selectivity for K2P Channels. ACS Chem. Neurosci. doi: 10.1021/acschemneuro.8b00337 [Epub ahead of print].
Poulin, H., Bruhova, I., Timour, Q., Theriault, O., Beaulieu, J. M., Frassati, D., et al. (2014). Fluoxetine blocks Nav1.5 channels via a mechanism similar to that of class 1 antiarrhythmics. Mol. Pharmacol. 86, 378–389. doi: 10.1124/mol.114.093104
Poupon, L., Lamoine, S., Pereira, V., Barriere, D. A., Lolignier, S., Giraudet, F., et al. (2018). Targeting the TREK-1 potassium channel via riluzole to eliminate the neuropathic and depressive-like effects of oxaliplatin. Neuropharmacology 140, 43–61. doi: 10.1016/j.neuropharm.2018.07.026
Rennolds, J., Tower, C., Musgrove, L., Fan, L., Maloney, K., Clancy, J. P., et al. (2008). Cystic fibrosis transmembrane conductance regulator trafficking is mediated by the COPI coat in epithelial cells. J. Biol. Chem. 283, 833–839. doi: 10.1074/jbc.M706504200
Rodrigues, N., Bennis, K., Vivier, D., Pereira, V., Chatelain, C. F., Chapuy, E., et al. (2014). Synthesis and structure-activity relationship study of substituted caffeate esters as antinociceptive agents modulating the TREK-1 channel. Eur. J. Med. Chem. 75, 391–402. doi: 10.1016/j.ejmech.2014.01.049
Royal, P., Andres-Bilbe, A., Avalos Prado, P., Verkest, C., Wdziekonski, B., Schaub, S., et al. (2019). Migraine-associated TRESK mutations increase neuronal excitability through alternative translation initiation and inhibition of TREK. Neuron 101:e236. doi: 10.1016/j.neuron.2018.11.039
Sandoz, G., Tardy, M. P., Thummler, S., Feliciangeli, S., Lazdunski, M., and Lesage, F. (2008). Mtap2 is a constituent of the protein network that regulates twik-related K+ channel expression and trafficking. J. Neurosci. 28, 8545–8552. doi: 10.1523/JNEUROSCI.1962-08.2008
Sandoz, G., Thummler, S., Duprat, F., Feliciangeli, S., Vinh, J., Escoubas, P., et al. (2006). AKAP150, a switch to convert mechano-, pH- and arachidonic acid-sensitive TREK K(+) channels into open leak channels. EMBO J. 25, 5864–5872. doi: 10.1038/sj.emboj.7601437
Santos, R., Ursu, O., Gaulton, A., Bento, A. P., Donadi, R. S., Bologa, C. G., et al. (2017). A comprehensive map of molecular drug targets. Nat. Rev. Drug Discov. 16, 19–34. doi: 10.1038/nrd.2016.230
Sauter, D. R., Sorensen, C. E., Rapedius, M., Bruggemann, A., and Novak, I. (2016). pH-sensitive K(+) channel TREK-1 is a novel target in pancreatic cancer. Biochim. Biophys. Acta 1862, 1994–2003. doi: 10.1016/j.bbadis.2016.07.009
Schewe, M., Nematian-Ardestani, E., Sun, H., Musinszki, M., Cordeiro, S., Bucci, G., et al. (2016). A Non-canonical Voltage-Sensing Mechanism Controls Gating in K2P K(+) Channels. Cell 164, 937–949. doi: 10.1016/j.cell.2016.02.002
Shi, D. N., Yuan, Y. T., Ye, D., Kang, L. M., Wen, J., and Chen, H. P. (2018). MiR-183-5p alleviates chronic constriction injury-induced neuropathic pain through inhibition of TREK-1. Neurochem. Res. 43, 1143–1149. doi: 10.1007/s11064-018-2529-4
Song, J. H., Huang, C. S., Nagata, K., Yeh, J. Z., and Narahashi, T. (1997). Differential action of riluzole on tetrodotoxin-sensitive and tetrodotoxin-resistant sodium channels. J. Pharmacol. Exp. Ther. 282, 707–714.
Spillane, J., Kullmann, D. M., and Hanna, M. G. (2016). Genetic neurological channelopathies: molecular genetics and clinical phenotypes. J. Neurol. Neurosurg. Psychiatry 87, 37–48. doi: 10.1136/jnnp-2015-311233
Takahira, M., Sakurai, M., Sakurada, N., and Sugiyama, K. (2005). Fenamates and diltiazem modulate lipid-sensitive mechano-gated 2P domain K(+) channels. Pflugers Arch. 451, 474–478. doi: 10.1007/s00424-005-1492-5
Talley, E. M., Solorzano, G., Lei, Q., Kim, D., and Bayliss, D. A. (2001). Cns distribution of members of the two-pore-domain (KCNK) potassium channel family. J. Neurosci. 21, 7491–7505. doi: 10.1523/JNEUROSCI.21-19-07491.2001
Terrenoire, C., Lauritzen, I., Lesage, F., Romey, G., and Lazdunski, M. (2001). A TREK-1-like potassium channel in atrial cells inhibited by beta-adrenergic stimulation and activated by volatile anesthetics. Circ. Res. 89, 336–342. doi: 10.1161/hh1601.094979
Tertyshnikova, S., Knox, R. J., Plym, M. J., Thalody, G., Griffin, C., Neelands, T., et al. (2005). BL-1249 [(5,6,7,8-tetrahydro-naphthalen-1-yl)-[2-(1H-tetrazol-5-yl)-phenyl]-amine]: a putative potassium channel opener with bladder-relaxant properties. J. Pharmacol. Exp. Ther. 313, 250–259. doi: 10.1124/jpet.104.078592
Thummler, S., Duprat, F., and Lazdunski, M. (2007). Antipsychotics inhibit TREK but not TRAAK channels. Biochem. Biophys. Res. Commun. 354, 284–289. doi: 10.1016/j.bbrc.2006.12.199
Tong, L., Cai, M., Huang, Y., Zhang, H., Su, B., Li, Z., et al. (2014). Activation of K(2)P channel-TREK1 mediates the neuroprotection induced by sevoflurane preconditioning. Br. J. Anaesth. 113, 157–167. doi: 10.1093/bja/aet338
Unudurthi, S. D., Wu, X., Qian, L., Amari, F., Onal, B., Li, N., et al. (2016). Two-Pore K+ Channel TREK-1 regulates sinoatrial node membrane excitability. J. Am. Heart Assoc. 5:e002865. doi: 10.1161/JAHA.115.002865
Veale, E. L., Al-Moubarak, E., Bajaria, N., Omoto, K., Cao, L., Tucker, S. J., et al. (2014). Influence of the N terminus on the biophysical properties and pharmacology of TREK1 potassium channels. Mol. Pharmacol. 85, 671–681. doi: 10.1124/mol.113.091199
Veyssiere, J., Moha Ou Maati, H., Mazella, J., Gaudriault, G., Moreno, S., Heurteaux, C., et al. (2015). Retroinverso analogs of spadin display increased antidepressant effects. Psychopharmacology 232, 561–574. doi: 10.1007/s00213-014-3683-2
Viatchenko-Karpinski, V., Ling, J., and Gu, J. G. (2018). Characterization of temperature-sensitive leak K(+) currents and expression of TRAAK, TREK-1, and TREK2 channels in dorsal root ganglion neurons of rats. Mol. Brain 11:40. doi: 10.1186/s13041-018-0384-5
Vierra, N. C., Dadi, P. K., Jeong, I., Dickerson, M., Powell, D. R., and Jacobson, D. A. (2015). Type 2 diabetes–associated K + channel TALK-1 modulates β-cell electrical excitability, second-phase insulin secretion, and glucose homeostasis. Diabetes 64, 3818–3828. doi: 10.2337/db15-0280
Vivier, D., Soussia, I. B., Rodrigues, N., Lolignier, S., Devilliers, M., Chatelain, F. C., et al. (2017). Development of the first two-pore domain potassium channel TWIK-related K(+) channel 1-selective agonist possessing in vivo antinociceptive activity. J. Med. Chem. 60, 1076–1088. doi: 10.1021/acs.jmedchem.6b01285
Wang, M., Song, J., Xiao, W., Yang, L., Yuan, J., Wang, W., et al. (2012). Changes in lipid-sensitive two-pore domain potassium channel TREK-1 expression and its involvement in astrogliosis following cerebral ischemia in rats. J. Mol. Neurosci. 46, 384–392. doi: 10.1007/s12031-011-9598-z
Wang, W., Liu, D., Xiao, Q., Cai, J., Feng, N., Xu, S., et al. (2018). Lig4-4 selectively inhibits TREK-1 and plays potent neuroprotective roles in vitro and in rat MCAO model. Neurosci. Lett. 671, 93–98. doi: 10.1016/j.neulet.2018.02.015
Wang, W., Zhang, M., Li, P., Yuan, H., Feng, N., Peng, Y., et al. (2013). An increased TREK-1-like potassium current in ventricular myocytes during rat cardiac hypertrophy. J. Cardiovasc. Pharmacol. 61, 302–310. doi: 10.1097/FJC.0b013e318280c5a9
Weckhuysen, S., Mandelstam, S., Suls, A., Audenaert, D., Deconinck, T., Claes, L. R., et al. (2012). KCNQ2 encephalopathy: emerging phenotype of a neonatal epileptic encephalopathy. Ann. Neurol. 71, 15–25. doi: 10.1002/ana.22644
Wong, D. T., Perry, K. W., and Bymaster, F. P. (2005). Case history: the discovery of fluoxetine hydrochloride (Prozac). Nat. Rev. Drug Discov. 4, 764–774. doi: 10.1038/nrd1821
Woo, D. H., Han, K. S., Shim, J. W., Yoon, B. E., Kim, E., Bae, J. Y., et al. (2012). TREK-1 and Best1 channels mediate fast and slow glutamate release in astrocytes upon GPCR activation. Cell 151, 25–40. doi: 10.1016/j.cell.2012.09.005
Wu, X., Liu, Y., Chen, X., Sun, Q., Tang, R., Wang, W., et al. (2013). Involvement of TREK-1 activity in astrocyte function and neuroprotection under simulated ischemia conditions. J. Mol. Neurosci. 49, 499–506. doi: 10.1007/s12031-012-9875-5
Xian Tao, L., Dyachenko, V., Zuzarte, M., Putzke, C., Preisig-Muller, R., Isenberg, G., et al. (2006). The stretch-activated potassium channel TREK-1 in rat cardiac ventricular muscle. Cardiovasc. Res. 69, 86–97. doi: 10.1016/j.cardiores.2005.08.018
Xu, X., Pan, Y., and Wang, X. (2004). Alterations in the expression of lipid and mechano-gated two-pore domain potassium channel genes in rat brain following chronic cerebral ischemia. Brain Res. Mol. Brain Res. 120, 205–209. doi: 10.1016/j.molbrainres.2003.09.020
Ye, D., Li, Y., Zhang, X., Guo, F., Geng, L., Zhang, Q., et al. (2015). TREK1 channel blockade induces an antidepressant-like response synergizing with 5-HT1A receptor signaling. Eur. Neuropsychopharmacol. 25, 2426–2436. doi: 10.1016/j.euroneuro.2015.09.007
Zhou, M., Xu, G., Xie, M., Zhang, X., Schools, G. P., Ma, L., et al. (2009). TWIK-1 and TREK-1 are potassium channels contributing significantly to astrocyte passive conductance in rat hippocampal slices. J. Neurosci. 29, 8551–8564. doi: 10.1523/JNEUROSCI.5784-08.2009
Keywords : TREK-1, potassium, ion channel, K 2P , modulators, neurological disorders, arrhythmia
Citation: Djillani A, Mazella J, Heurteaux C and Borsotto M (2019) Role of TREK-1 in Health and Disease, Focus on the Central Nervous System. Front. Pharmacol. 10:379. doi: 10.3389/fphar.2019.00379
Received: 14 January 2019; Accepted: 26 March 2019; Published: 11 April 2019.
Reviewed by:
Copyright © 2019 Djillani, Mazella, Heurteaux and Borsotto. This is an open-access article distributed under the terms of the Creative Commons Attribution License (CC BY) . The use, distribution or reproduction in other forums is permitted, provided the original author(s) and the copyright owner(s) are credited and that the original publication in this journal is cited, in accordance with accepted academic practice. No use, distribution or reproduction is permitted which does not comply with these terms.
*Correspondence: Alaeddine Djillani, [email protected] ; [email protected]
† Present address: Alaeddine Djillani, Department of Pharmacology, University of Washington, Seattle, WA, United States
Disclaimer: All claims expressed in this article are solely those of the authors and do not necessarily represent those of their affiliated organizations, or those of the publisher, the editors and the reviewers. Any product that may be evaluated in this article or claim that may be made by its manufacturer is not guaranteed or endorsed by the publisher.
Trek Bikes - The world's best bikes and cycling gear
Online bike shopping made easy
Your perfect bike may already be in a store near you. Browse local inventory online for the fastest availability.
Upon arrival, your bike will be assembled by certified bicycle technicians and made ready to pick up or deliver to your home.
If you’re not in love with your bike within the first thirty days, we will take it back without any additional charges or hassle.
Your local bike shop has the knowledge, products, and expertise to help you get the most out of your new bike.
Back-to-back bike of the year
Slash - 2023 Bike of the Year
Fuel ex - 2022 bike of the year.
We’re a different kind of bike company
Used bikes, certified awesome by Trek
Red Barn Refresh, where pre-owned bikes get another chance to ride.
No time to waste
We’re changing the business of bikes
Trek is a 2024 Fortune 100 Best Company to Work For!
Looking for a great place to work.
Our mission Trek started in a small Wisconsin barn in 1976, but our founders always saw something bigger. Decades later, we're on a mission to make our world a better place to live and ride. We build only products we love, provide incredible hospitality to our customers, and change the world by getting more people on bikes.
Star Trek: The Next Generation Season 1 Streaming: Watch & Stream Online via Paramount Plus
A re you wondering where to stream Star Trek: The Next Generation Season 1 ? Then scroll ahead to find that answer below. Premiered back in 1987, the sci-fi, fantasy-adventure series revolves around Captain Jean-Luc Picard who forms a new generation of Starfleet to explore new planets and possibilities.
Here’s how you can watch and stream Star Trek: The Next Generation Season 1 via streaming services such as Paramount Plus.

Is Star Trek: The Next Generation Season 1 available to watch via streaming?
Yes, Star Trek: The Next Generation Season 1 is available to watch via streaming on Paramount Plus.
After Star Trek: The Originals, Star Trek: The Next Generation was made as a spin-off. Created by Gene Roddenberry, the sci-fi series follows Captain Jean-Luc Picard and his loyal group aboard the USS Enterprise NCC-1701D to explore new worlds. This American science fiction television series focuses on new advancements and possibilities. With every invention, they show how they face new challenges.
Star Trek: The Next Generation has a stellar star cast. From Patrick Stewart, Jonathan Frakes, Michael Dorn, Gates McFadden, Marina Sirtis, and more.
Watch Star Trek: The Next Generation Season 1 streaming via Paramount Plus
Star Trek: The Next Generation Season 1 i s available to watch on Paramount Plus.
Owned by Paramount World, Paramount Plus is an American subscription-based streaming service.
You can watch via Paramount Plus by following these steps:
- Go to ParamountPlus.com
- Select ‘Try It Free’
- $5.99 per month or $59.99 per year (Essential)
- $11.99 per month or $199.99 per year (with SHOWTIME)
- Enter your personal information and create your account
The Paramount Plus Essential plan includes tens of thousands of episodes and movies, the NFL on CBS, the UEFA Champions League, 24/7 news coverage with CBS News, and limited ads.
Furthermore, the Paramount Plus with SHOWTIME plan includes all of the above, removes the ads except in limited circumstances, and also includes SHOWTIME originals, movies, and sports along with CBS live TV and college football. Nonetheless, you’re able to download shows to your mobile device.
Star Trek: The Next Generation’s synopsis is as follows:
“Follow the intergalactic adventures of Capt. Jean-Luc Picard and his loyal crew aboard the all-new USS Enterprise NCC-1701D, as they explore new worlds.”
NOTE: The streaming services listed above are subject to change. The information provided was correct at the time of writing.
Jeremy Renner Revealed He ‘Died’ After Snowplow Accident, Claims Co-Star
The hangman trailer brings backwoods horror to screens this month, mario beats barbie as most profitable movie of 2023, diary of the dead blu-ray steelbook release date set for george romero zombie movie.
The post Star Trek: The Next Generation Season 1 Streaming: Watch & Stream Online via Paramount Plus appeared first on ComingSoon.net - Movie Trailers, TV & Streaming News, and More .
Screen Rant
Burnham & tilly’s star trek: discovery away mission is a brilliant season 1 callback.

Your changes have been saved
Email Is sent
Please verify your email address.
You’ve reached your account maximum for followed topics.
Star Trek: Discovery's Mary Wiseman On Tilly's Season 5 Arc & Burnham Friendship
I've been missing detmer & owosekun in star trek: discovery season 5, star trek: discovery’s new vulcan name ni’var is a deep cut enterprise callback.
WARNING: Contains SPOILERS for Star Trek: Discovery, season 5, episode 6, "Whistlespeak".
- Captain Burnham and Lt. Tilly face the challenging Race of the Mother Compeer on pre-warp planet Halem'no.
- Running the race together builds trust between Burnham and Tilly, mirroring their bond from season 1.
- Lt. Tilly abandons her dream of becoming a Starfleet captain to pursue a new path as a Starfleet Academy instructor.
There's a brilliant callback to Star Trek: Discovery season 1 in the away mission to pre-warp planet Halem'no in Star Trek: Discovery season 5, episode 6 , "Whistlespeak", written by Kenneth Lin and Brandon Schultz, and directed by Chris Byrne. Captain Michael Burnham (Sonequa Martin-Green) selects Lieutenant Sylvia Tilly (Mary Wiseman) to join her in retrieving the next clue in Star Trek: Discovery season 5's race for the Progenitors' technology, which is hidden in one of Halem'no's faulty weather towers. The only catch is that the people of Halem'no consider the towers to be sacred temples, and entry is only permitted after winning a harrowing race.
Few Halem'nites actually finish The Race of the Mother Compeer because completing the race relies on stamina and constitution more than speed, but Captain Burnham and Sylvia Tilly, with their Starfleet training, are up to the task. The race is a difficult undertaking that recreates a Halem'no legend in which the Mother Compeer traversed Halem'no's arid deserts to procure water for her children. To better replicate the Mother Compeer's environmental and physical conditions, contestants ingest a cube that induces dehydration and are then expected to complete the course without a single drop of water. Many Halem'nites give in to temptation, but Tilly doesn't.
Screen Rant interviews Mary Wiseman about Star Trek: Discovery season 5, Tilly's friendship with Burnham, and her thoughts on the series ending.
Burnham & Tilly’s Away Mission Is A Star Trek: Discovery Season 1 Callback
Burnham & tilly bonded over running together.
The sight of Captain Michael Burnham and Lieutenant Sylvia Tilly running the Race of the Mother Compeer together in Star Trek: Discovery season 5, episode 6, "Whistlespeak", calls back to when Specialist Burnham helps Cadet Tilly prepare for Starfleet's Command Training program in Star Trek: Discovery season 1. When Burnham and Tilly first meet as roommates in Star Trek: Discovery season 1, episode 3, "Context is For Kings", Tilly is a nervous cadet determined to become a Starfleet Captain someday. Having been through Starfleet's Command Training Program, Michael recognizes Tilly needs an advantage to be selected, and she becomes Tilly's personal trainer in Star Trek: Discovery season 1, episode 6, "Lethe".
Running together also builds trust between Michael Burnham and Sylvia Tilly.
Burnham and Tilly's races through the USS Discovery corridors wearing "DISCO" -branded athletic gear throughout Star Trek: Discovery season 1 are part of Tilly's physical endurance training. But running together also builds trust between Michael Burnham and Sylvia Tilly, who become one of Star Trek: Discovery 's best friendships . Burnham's mentorship also helps Tilly's confidence , which, in turn, results in Commander Saru (Doug Jones) providing the command-level recommendation Tilly needs to apply to Starfleet's Command Training Program. In Star Trek: Discovery season 1, episode 15, "Will You Take My Hand?", Sylvia Tilly becomes the youngest person accepted for the Command Training Program, but Tilly never completes the course.
Why Tilly Abandoned Her Captain Dream To Become A Starfleet Academy Teacher Instead
Lt. tilly gets a fresh start in star trek: discovery's 32nd century.
Lt. Sylvia Tilly abandons her dream of becoming a Starfleet captain and chooses to be a Starfleet Academy instructor after Tilly realizes pursuing command isn't really what she wants for herself. Being named Captain Saru's Number One in Star Trek: Discovery season 3 helped Ensign Tilly determine that becoming a Starfleet Captain was actually just a way to prove to Tilly's overly critical mother, Federation diplomat Siobhan Tilly (Mimi Kuzyk), that Sylvia was truly capable of succeeding in Starfleet. Without the need to justify a Starfleet career with prestigious goals, Tilly leaves the pressure of attaining Siobhan's approval 900 years in the past, and drops the Command Training Program.
Sylvia Tilly's mother, Siobhan, appears in Star Trek: Short Treks season 1, episode 1, "Runaway", when Sylvia speaks with Siobhan via hologram.
A whole galaxy of possibilities is available in the future of the 32nd century, but one of Lieutenant Tilly's away missions training Starfleet Academy cadets marks a huge turning point for her. Despite -- or perhaps because of -- the disaster that befalls the cadets' shuttle in Star Trek: Discovery season 4, episode 3, "All Is Possible", Tilly teaches cadets to cooperate in deadly circumstances, and decides to become a Starfleet Academy instructor. Teaching at the Academy is the perfect path for Tilly, but her physical endurance training with Michael Burnham still pays off in Star Trek: Discovery season 5, when Tilly completes and wins the Race of the Mother Compeer.
Star Trek: Discovery season 5 streams Thursdays on Paramount+.
Star Trek: Discovery

Star Trek's Top Exec Has One Strict Rule For Any Future Shows
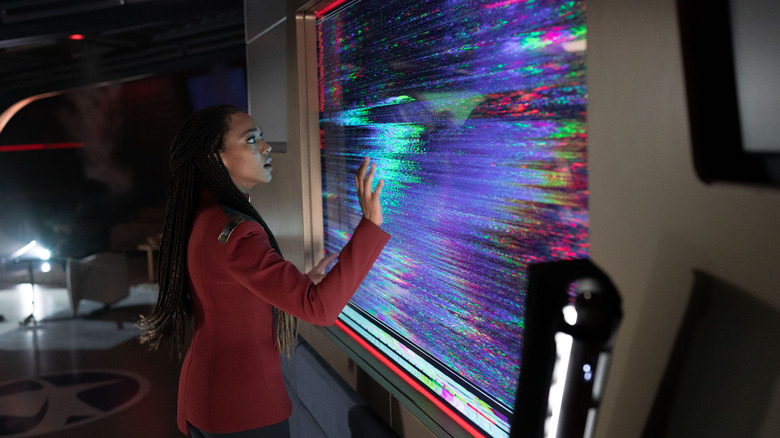
Since 2015, screenwriter and producer Alex Kurtzman has been the head honcho of "Star Trek" over at Paramount. Although Bryan Fuller came up with the initial idea for the streaming era's inaugural "Star Trek" series "Star Trek: Discovery," which debuted in 2017, it was Kurtzman who completely reworked the show from its origins, hence launching Paramount+ as an all-our-eggs-in-the-"Star"-"Trek"-franchise's-basket service. Kurtzman served as executive producer on all five "Star Trek" shows that began after "Discovery," including the now-concluded "Star Trek: Short Treks" and "Star Trek: Picard," as well as the soon-to-be-concluded "Star Trek: Prodigy" and "Star Trek: Lower Decks." Also soon to conclude is "Discovery" itself, which is currently partway through its fifth and final season.
As of this writing, "Star Trek: Strange New Worlds" is the only series that hasn't been definitively canceled, while the "Section 31" TV film has yet to stream. Also in the works is a "Starfleet Academy" series that is still in the early days of its production. Given the financial woes over at Paramount and the destruction wrought by the Streaming Wars, the future of "Star Trek" will most certainly be modest, if not downright austere. Apologies to the people who eagerly wanted to see the proposed "Star Trek: Legacy," a hyped potential spinoff from "Picard." That series will likely never be made.
On the "Star Trek" recap chat show "The Ready Room," hosted by Wil Wheaton, Kurtzman was asked about the future of "Star Trek," and the exec was, perhaps predictably, diplomatic. He couldn't very well say that that franchise was on fire, or that Paramount was actively taking a step back from one of its most lucrative properties, but he did say that he would follow a strict rule for any new "Star Trek" shows: there needs to be something to say.
The glut of Star Trek
Kurtzman admitted that he was just following the whims of creativity (and of the entertainment marketplace) when he started overseeing more and more "Star Trek" from 2017 to 2021, saying:
"[T]he truth is that when we started 'Discovery,' I wasn't thinking about that. I was just thinking about making a great show. And I fell so deeply in love with 'Discovery' and with the process of making it. I spent a lot of time that first season really kind of meditating on this incredible universe that has been around for so long, and how much more can be done with it; how many amazing stories there are to tell. There's really an infinite number of stories to tell."
One might argue that Kutrzman's stories are hit-and-miss. "Discovery" has just as many detractors as fans, and many agree that "Picard" only had one good season among its three (and even then, complaints can be made about "the good one"). One cannot deny, however, that the stories have been plentiful. Between the six shows that Kurtzman has overseen, there have been 210 episodes, with a promised 20 more on the way. If that number of episodes were applied to 1990s "Star Trek," it would equal almost nine full seasons in only six-and-a-half years. Never before, not even in its '90s heyday, did "Star Trek" produce the same sheer volume.
When moving forward, however, Kurtzman said he wanted the franchise to be about quality and not quantity. He may claim to have infinite stories, but there aren't, he said, infinite themes to dissect. Each potentially new "Star Trek" series, he feels, needs to have a point.
Enough of quantity, Star Trek needs more quality
In Kurtzman's own words:
"I only want to make another 'Star Trek' show if there's something special to say. I don't want to do it just to do more. I think that would be a huge disservice to 'Star Trek.' It would be a huge disservice to the fans. I think the fans would feel it instantaneously. They would know this is not authentic because if there's one thing that 'Trek' fans know, it's authenticity. So for me, it's really just about figuring out a way to make sure that we take our time, we're deliberate, we're thoughtful, and we deliver on the promise of something different every time."
Some of the harsher "Discovery" critics might immediately chime in, pleading that Kurtzman should have taken on that attitude seven years ago. Sadly, Kurtzman does not elucidate on what the current spate of "Star Trek" shows has been saying; it would have been nice if he gave a thematic rundown on how each individual show is about something specific. "Discovery," for instance, is about emotional turmoil and how workmates cling to each other for warmth. "Picard" is about how one frets over their legacy. "Lower Decks" is about workplace camaraderie and the quarter-life crisis, "Prodigy" is about maturity, and "Strange New Worlds" is about whimsy in the face of terror.
Kurtzman also doesn't mention what "Section 31" or "Starfleet Academy" might say.
Perhaps, however, Kurtzman wishes any future "Star Trek" shows to break a trend. Perhaps he feels that "Star Trek" hasn't been communicating enough in terms of philosophy, themes, and ideas, choosing instead to fall back on the bland efficiencies of storytelling. If we're to get more "Star Trek," like he said, we should expect more.
More From Forbes
‘star trek: discovery’ co-showrunner teases the final episodes and her message for fans.
- Share to Facebook
- Share to Twitter
- Share to Linkedin
Co-showrunner Michelle Paradise with her "Star Trek: Discovery" cast and crew.
For five seasons, Star Trek: Discovery has taken audiences on the ride of a lifetime. With its series finale now in sight, I sat down with executive producer and co-showrunner Michelle Paradise at SeriesFest in Denver, Colorado to discuss the beloved show’s journey and what fans should expect from this ever-expanding television universe on Paramount Plus, before we say a proper farewell to the U.S.S. Discovery.
So for starters, what has Paradise enjoyed most about the evolution of Star Trek: Discovery , since it first premiered in September 2017?
“That’s a really great question,” Paradise said. “I have loved being a part of it. I joined halfway through season two, and it was starting to know what it was and it’s really found its way. I’ve really enjoyed the character arcs and getting to take them all to these different places. It’s also hard for me to separate out the evolution of the show from my evolution on the show and stepping into co-running it with [co-showrunner] Alex [Kurtzman] and starting to take over more of that in later seasons. It’s been the highlight of my career.”
Mary Wiseman as Tilly and Blu del Barrio as Adira in "Star Trek: Discovery" episode 7, season 5 - ... [+] streaming on Paramount+.
Paradise is no stranger to television production. She was previously an executive producer on such television projects as Exes & Ohs and The Originals , but I wondered what it might be about her Star Trek: Discovery cast and crew that perhaps feels special.
“It’s like a big family. First of all, our cast is incredible. They’re all insanely talented actors but they’re lovely human beings. They approach one another and they approach the project from just a place of love and respect for one another and for the material, which is always a wonderful place to start. You can’t say enough of [actor] Sonequa [Martin-Green], our number one and the tone that she sets on the show and on the set. We have a lot of folks on the show and the crew who come back, year-after-year. They all really love the world of Trek and they love one another. We try and go bigger and better and bolder every season, and there’s never a feeling of Oh, we can’t do that . It’s always a feeling of How do we do that? ”
New FBI Warning As Hackers Strike: Email Senders Must Do This 1 Thing
2 obvious signs of workplace gaslighting from a psychologist, wells fargo championship 2024 golf betting preview odds and pga picks.
Sonequa Martin-Green as Burnham in "Star Trek: Discovery" streaming on Paramount+.
Since the original Star Trek series first premiered on television in 1966, the Star Trek story and fictional sci-fi universe has evolved into several iterations, including Star Trek: The Next Generation , Deep Space Nine , Voyager , Enterprise , Strange New Worlds and Picard - not to mention the numerous Star Trek movies over many decades, including the three recent blockbuster films, starring Chris Pine, Zoe Saldana and Zachary Quinto, with another sequel in the works.
Throughout all of these years, Star Trek fans have remained quite loyal and vocal, both when they love a story and even when they don’t. So, how does Paradise and her Star Trek: Discovery creative team try to appease Trek fans, but also not allow the chatter to stop them from taking on some creative risks?
Wilson Cruz as Culber and Anthony Rapp as Stamets in "Star Trek: Discovery" episode 6, season 5 - ... [+] streaming on Paramount+.
Paradise said, “I think the best way to answer that is we always try to honor what Trek is - where it came from, what is the DNA of any Trek series, which of course goes back to the original series, and then also honoring what is this version of Trek and knowing that it’s our job to make the best version of Discovery . Someone who loves Discovery may not love Strange New Worlds or someone who loves Strange New Worlds may not love Discovery. By design, these are all very different shows. To a certain degree, it has to be okay that not everyone is going to love this iteration. We always feel like if we can do justice to the franchise as a whole - what it means, do justice to the characters and do our best version of Discovery , then we will have succeeded.”
Ever since the WGA and SAG-AFTRA strikes ended late last year, there have been signs of movement and optimism within the entertainment industry, but also a sense of some standstill with television and film productions. So, what is Paradise seeing around the current state of Hollywood?
“I think it depends on who you talk to,” Paradise said. “It feels a bit like a mixed bag to me. There are a lot of people who are still very much struggling - writers, crew members, directors - because things have not picked up in the way that they would normally at this particular time of year. By in large, there’s not really a pilot season, as we used to know it. That’s very difficult for people. Of course, there’s still a lot of wonderful opportunities, but it seems like the compression that happened - it’s the combo platter of the [Covid-19] pandemic and the strikes, just after that. It does feel condensed - there are fewer things but I feel like, to some degree, that had to happen because in the big balloon of streaming, that in retrospect, it doesn’t feel like it was necessarily sustainable. Hopefully it will ease up, sooner than later, and kind of calm down because there’s still a lot of people who are struggling to get back into work right now and I feel for them - it’s hard.”
On more of a positive note, when asked what Paradise is enjoying most about the television landscape today, she says that she feels there is a lot that can be done, applauding the way that every week, Star Trek: Discover y is a “cinematic storytelling into television,” though she admits that the visual design of Discovery is not sustainable for every network and studio.
While our real world faces divisive times with war, political unrest and other conflicts, I wondered if Paradise and her team keep in mind the real-life issues occurring, when crafting its stories for the screen on Star Trek: Discovery .
Mary Wiseman as Tilly, June Laporte as Ravah and Wilson Cruz as Dr. Culber in "Star Trek: Discovery" ... [+] episode 6, season 5 - streaming on Paramount+.
“That comes from just the DNA of the show itself. The original series did not shy away from what was happening societal at that time. It did not shy away from tackling those things in the way that it could. That’s one of the cool things about sci-fi - aliens can stand-in for another culture, different relationships can stand-in for whatever is happening in society at the time. That’s kind of baked into any Trek show, is that reflection of what’s happening in the world at that time via sci-fi and the different ways we could do it.”
Paradise added: “In Discovery , we were very much aware of that and very thoughtful about what are the types of stories we want to tell, how does that reflect certain things that are going on. Trek means so much to so many people and where people are actively looking to Trek to reflect themselves in some way or actively looking to Trek as an answer to what’s happening in society and for hope that, as divisive as things are right now, as challenging around the world and in our country, that there is hope and we can overcome it with our better selves.”
Blu del Barrio as Adira, Tig Notaro as Jett Reno and Mary Wiseman as Tilly in "Star Trek: Discovery" ... [+] episode 7, season 5 - streaming on Paramount+.
When asked about what fans can expect ahead in the remaining episodes of Star Trek: Discovery , which drop every Thursday during this final season, Paradise teases that fans “might see more of our bad guys this season.” She added: “We did not going into the season [knowing] that this would be our last season. We’re so fortunate - CBS Studios and Paramount Plus gave us the opportunity, after the fact, to go shoot some additional material, and I feel like the thing I just want to keep reiterating for fans of the show is it has a proper ending. Our Discovery is not going to end on a cliffhanger of Are they all going to die? and then we never find out. It was worth the investment and I do think when people finish the season/series, they will feel like this season - again, we didn’t know going in - but it does feel like if you didn’t know that, you would think we knew going in because of what we’re doing thematically and where our characters go. So, I think people will feel very satisfied.”
As the conversation with Paradise concluded, she was left with one last question: For Star Trek: Discovery fans, people who have followed these five seasons - as they soon finish these final episodes, what message do you as a co-showrunner want to say these people, after investing their time in the series over the past seven years and falling in love with these characters?
Mary Wiseman, Sonequa Martin-Green and Michelle Paradise.
“I just would want to say thank you. There is no Discovery without the fans and they are always so loving and supportive and passionate, even when they don’t like what we’re doing, they’re passionate. As writers and creators and actors and the directors, we love that! We love that passion and their passion means so much to us.”
Paradise added: “It was a gift. Hopefully, we’ll all get to do it again someday.”
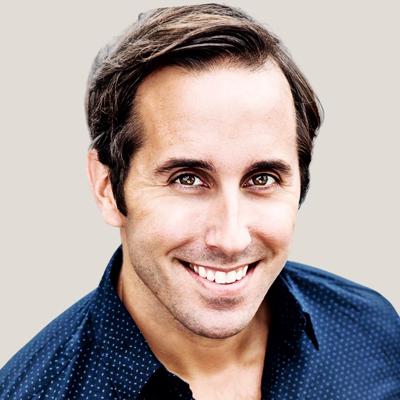
- Editorial Standards
- Reprints & Permissions
Russia Travel Blog | All about Russia in English
- About our blog
- RussiaTrek.org
Sidebar →
- Architecture
- Entertainment
- RussiaTrek.org News
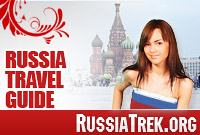
- Send us a tip with a message
- Support RussiaTrek.org
- Travel Guide to Ukraine
- Comments RSS
← Sidebar
The trains and stations of the Moscow Metro
2 Comments · Posted by Alex Smirnov in Cities , Travel , Video
The Moscow Metro is the third most intensive subway system in the world after Tokyo and Seoul subways. The first line was opened on May 15, 1935. Since 1955, the metro has the name of V.I. Lenin.
The system consists of 12 lines with a total length of 305.7 km. Forty four stations are recognized cultural heritage. The largest passenger traffic is in rush hours from 8:00 to 9:00 and from 18:00 to 19:00.
Cellular communication is available on most of the stations of the Moscow Metro. In March 2012, a free Wi-Fi appeared in the Circle Line train. The Moscow Metro is open to passengers from 5:20 to 01:00. The average interval between trains is 2.5 minutes.
The fare is paid by using contactless tickets and contactless smart cards, the passes to the stations are controlled by automatic turnstiles. Ticket offices and ticket vending machines can be found in station vestibules.
Tags: Moscow city
You might also like:
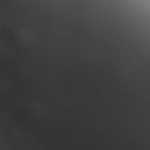
The bridge over Zolotoy Rog Bay in Vladivostok
The views of St. Petersburg from the TV tower >>
Tomás · August 27, 2012 at 11:34 pm
The Moscow metro stations are the best That I know, cars do not.
Alberto Calvo · September 25, 2016 at 8:57 pm
Great videos! Moscow Metro is just spectacular. I actually visited Moscow myself quite recently and wrote a post about my top 7 stations, please check it out and let me know what you think! :)
http://www.arwtravels.com/blog/moscow-metro-top-7-stations-you-cant-miss
Leave a Reply
XHTML: You can use these tags: <a href="" title=""> <abbr title=""> <acronym title=""> <b> <blockquote cite=""> <cite> <code> <del datetime=""> <em> <i> <q cite=""> <s> <strike> <strong>
- February 2024
- January 2024
- December 2023
- November 2023
- October 2023
- September 2023
- August 2023
TREK-1 protects the heart against ischemia-reperfusion-induced injury and from adverse remodeling after myocardial infarction
Affiliations.
- 1 Department of Physiology, University of Tennessee Health Sciences Center, 71 S. Manassas Street, Memphis, TN, 38163, USA.
- 2 Department of Physiology, Saha Cardiovascular Research Center, University of Kentucky, Lexington, KY, USA.
- 3 Department of Pediatrics, University of California Los Angeles, Los Angeles, CA, USA.
- 4 Department of Physiology, University of Tennessee Health Sciences Center, 71 S. Manassas Street, Memphis, TN, 38163, USA. [email protected].
- PMID: 31511966
- PMCID: PMC8996126
- DOI: 10.1007/s00424-019-02306-y
The TWIK-related K+ channel (TREK-1) is a two-pore-domain potassium channel that produces background leaky potassium currents. TREK-1 has a protective role against ischemia-induced neuronal damage. TREK-1 is also expressed in the heart, but its role in myocardial ischemia-reperfusion (IR)-induced injury has not been examined. In the current study, we used a TREK-1 knockout (KO) mouse model to show that TREK-1 has a critical role in the cardiac I/R-induced injury and during remodeling after myocardial infarction (MI). At baseline, TREK-1 KO mice had similar blood pressure and heart rate as the wild-type (WT) mice. However, the lack of TREK-1 was associated with increased susceptibility to ischemic injury and compromised functional recovery following ex vivo I/R-induced injury. TREK-1 deficiency increased infarct size following permanent coronary artery ligation, resulting in greater systolic dysfunction than the WT counterpart. Electrocardiographic (ECG) analysis revealed QT interval prolongation in TREK-1 KO mice, but normal heart rate (HR). Acutely isolated TREK-1 KO cardiomyocytes exhibited prolonged Ca 2+ transient duration associated with action potential duration (APD) prolongation. Our data suggest that TREK-1 has a protective effect against I/R-induced injury and influences the post-MI remodeling processes by regulating membrane potential and maintaining intracellular Ca 2+ homeostasis. These data suggest that TREK-1 activation could be an effective strategy to provide cardioprotection against ischemia-induced damage.
Keywords: Ischemia-reperfusion; Mice; Myocardial infarction; Potassium channel; TREK-1; Telemetry.
Publication types
- Research Support, N.I.H., Intramural
- Action Potentials
- Blood Pressure
- Calcium Signaling
- Cells, Cultured
- Mice, Inbred C57BL
- Myocardial Reperfusion Injury / genetics
- Myocardial Reperfusion Injury / metabolism*
- Myocytes, Cardiac / metabolism
- Myocytes, Cardiac / physiology
- Potassium Channels, Tandem Pore Domain / genetics
- Potassium Channels, Tandem Pore Domain / metabolism*
- Potassium Channels, Tandem Pore Domain
- potassium channel protein TREK-1
Grants and funding
- R01 HL123540/HL/NHLBI NIH HHS/United States
- K99 HL114869/HL/NHLBI NIH HHS/United States
- HL114869/HL/NHLBI NIH HHS/United States
- R01 HL131526/HL/NHLBI NIH HHS/United States
- R00 HL114869/HL/NHLBI NIH HHS/United States
William Shatner Wants to Return to 'Star Trek,' But There's a Catch
Shatner hasn't starred in a 'Star Trek' story since 2006.
The Big Picture
- William Shatner expressed interest in returning as Captain Kirk, desiring a substantial role, not just a cameo.
- Shatner considers de-aging technology as a route to reprise his iconic role.
- AI technology in the entertainment industry continues to stir debate about job-cutting implications.
At 93 years young, beloved science fiction icon William Shatner has expressed interest in returning to the Star Trek franchise. Shatner's galaxy-renowned role as Captain James 'Tiberius' Kirk of the U.S.S. Enterprise has inspired countless stories in the Star Trek universe, but it's been quite a while since Shatner himself has been a part of any of those stories. However, in a recent interview with The Canadian Press , it seems William Shatner is willing to consider a grand return, even if it means going the de-aging route to bring Kirk back on screen.
While promoting his new documentary, You Can Call Me Bill , Shatner said in his Canadian Press interview that he found the idea of returning as Captain Kirk to be "intriguing". Shatner did also go on to say that, if he does return to his most iconic role, he doesn't want it to be a mere cameo. It's certainly the most positive Shatner has been about returning to the franchise in a long time, as the former lead has been openly critical of the franchise's modern direction in the past. He wants to be an active participant in a potential new story that feels warranted, which is further reiterated in the following statement:
“It’s almost impossible but it was a great role and so well-written and if there were a reason to be there not just to make a cameo appearance, but if there were a genuine reason for the character appearing, I might consider it.”
How Can William Shatner Return as Captain Kirk After 'Star Trek: Generations'?
Some may be wondering how the 94-year-old actor could return as Captain Kirk in a future Star Trek project, given how Shatner's character was seemingly killed off in Star Trek: Generations . As to that question, it seems Shatner has thought that through as well. Following the actor's spokesperson deal with AI specialist company Otoy, Shatner recommended that de-aging could be the answer , as the technology has the potential to make anyone "look 10, 20, 30, 50 years younger." It's an in-character response from the actor, given that Shatner has a history of being more open with the prospects of AI than others in the industry.
De-aging, deep faking, and AI continue to be contentious aspects of the entertainment industry. Not only is the technology still in its early days with even the most experienced filmmakers studying to harness it ( looking at you, The Irishman ), but the potential for job-cutting is one of the reasons the recent industry-wide strikes took place.
Shatner allowing the use of AI technology to bring back a beloved iconic character wouldn't be the first instance of that in the sci-fi world either, as Star Trek 's long-time rival franchise Star Wars recently got the exclusive rights to recreate James Earl Jones ' voice as Darth Vader for future projects. Shatner's last appearance as Captain Kirk was in the 2006 video game, Star Trek: Tactical Assault . His last on-screen appearance was Star Trek: Generations , and there's no doubt a large group of Trekkies who would love to see Shatner return to the role.
Whether we see Shatner return to Star Trek or not, you can get a new glimpse of the actor through the acclaimed documentary You Can Call Me Bill , which is now available to rent or buy on Prime Video . Watch the trailer below.
You Can Call Me Bill
Watch on Prime Video

40 Facts About Elektrostal
Written by Lanette Mayes
Modified & Updated: 02 Mar 2024

Reviewed by Jessica Corbett
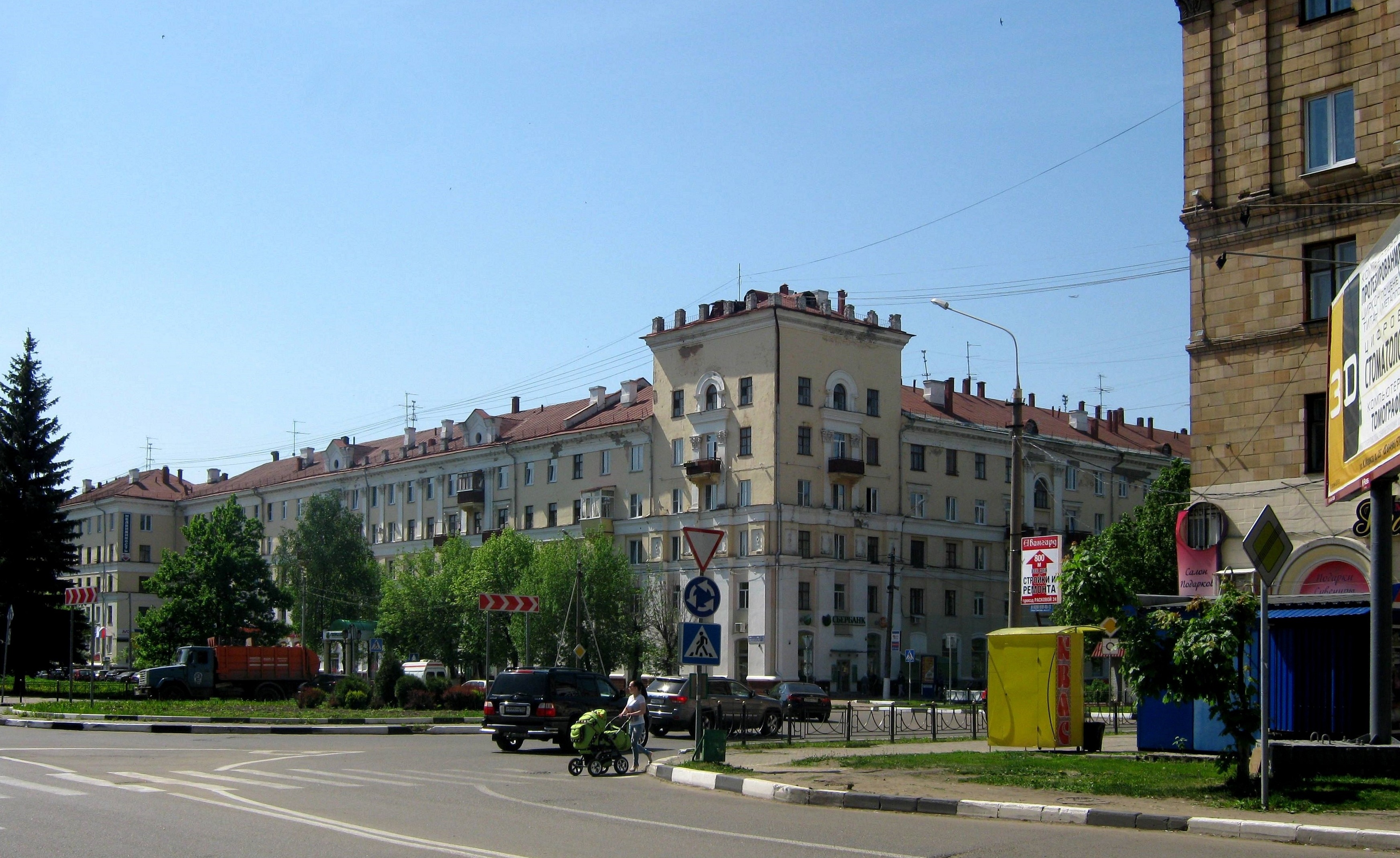
Elektrostal is a vibrant city located in the Moscow Oblast region of Russia. With a rich history, stunning architecture, and a thriving community, Elektrostal is a city that has much to offer. Whether you are a history buff, nature enthusiast, or simply curious about different cultures, Elektrostal is sure to captivate you.
This article will provide you with 40 fascinating facts about Elektrostal, giving you a better understanding of why this city is worth exploring. From its origins as an industrial hub to its modern-day charm, we will delve into the various aspects that make Elektrostal a unique and must-visit destination.
So, join us as we uncover the hidden treasures of Elektrostal and discover what makes this city a true gem in the heart of Russia.
Key Takeaways:
- Elektrostal, known as the “Motor City of Russia,” is a vibrant and growing city with a rich industrial history, offering diverse cultural experiences and a strong commitment to environmental sustainability.
- With its convenient location near Moscow, Elektrostal provides a picturesque landscape, vibrant nightlife, and a range of recreational activities, making it an ideal destination for residents and visitors alike.
Known as the “Motor City of Russia.”
Elektrostal, a city located in the Moscow Oblast region of Russia, earned the nickname “Motor City” due to its significant involvement in the automotive industry.
Home to the Elektrostal Metallurgical Plant.
Elektrostal is renowned for its metallurgical plant, which has been producing high-quality steel and alloys since its establishment in 1916.
Boasts a rich industrial heritage.
Elektrostal has a long history of industrial development, contributing to the growth and progress of the region.
Founded in 1916.
The city of Elektrostal was founded in 1916 as a result of the construction of the Elektrostal Metallurgical Plant.
Located approximately 50 kilometers east of Moscow.
Elektrostal is situated in close proximity to the Russian capital, making it easily accessible for both residents and visitors.
Known for its vibrant cultural scene.
Elektrostal is home to several cultural institutions, including museums, theaters, and art galleries that showcase the city’s rich artistic heritage.
A popular destination for nature lovers.
Surrounded by picturesque landscapes and forests, Elektrostal offers ample opportunities for outdoor activities such as hiking, camping, and birdwatching.
Hosts the annual Elektrostal City Day celebrations.
Every year, Elektrostal organizes festive events and activities to celebrate its founding, bringing together residents and visitors in a spirit of unity and joy.
Has a population of approximately 160,000 people.
Elektrostal is home to a diverse and vibrant community of around 160,000 residents, contributing to its dynamic atmosphere.
Boasts excellent education facilities.
The city is known for its well-established educational institutions, providing quality education to students of all ages.
A center for scientific research and innovation.
Elektrostal serves as an important hub for scientific research, particularly in the fields of metallurgy, materials science, and engineering.
Surrounded by picturesque lakes.
The city is blessed with numerous beautiful lakes, offering scenic views and recreational opportunities for locals and visitors alike.
Well-connected transportation system.
Elektrostal benefits from an efficient transportation network, including highways, railways, and public transportation options, ensuring convenient travel within and beyond the city.
Famous for its traditional Russian cuisine.
Food enthusiasts can indulge in authentic Russian dishes at numerous restaurants and cafes scattered throughout Elektrostal.
Home to notable architectural landmarks.
Elektrostal boasts impressive architecture, including the Church of the Transfiguration of the Lord and the Elektrostal Palace of Culture.
Offers a wide range of recreational facilities.
Residents and visitors can enjoy various recreational activities, such as sports complexes, swimming pools, and fitness centers, enhancing the overall quality of life.
Provides a high standard of healthcare.
Elektrostal is equipped with modern medical facilities, ensuring residents have access to quality healthcare services.
Home to the Elektrostal History Museum.
The Elektrostal History Museum showcases the city’s fascinating past through exhibitions and displays.
A hub for sports enthusiasts.
Elektrostal is passionate about sports, with numerous stadiums, arenas, and sports clubs offering opportunities for athletes and spectators.
Celebrates diverse cultural festivals.
Throughout the year, Elektrostal hosts a variety of cultural festivals, celebrating different ethnicities, traditions, and art forms.
Electric power played a significant role in its early development.
Elektrostal owes its name and initial growth to the establishment of electric power stations and the utilization of electricity in the industrial sector.
Boasts a thriving economy.
The city’s strong industrial base, coupled with its strategic location near Moscow, has contributed to Elektrostal’s prosperous economic status.
Houses the Elektrostal Drama Theater.
The Elektrostal Drama Theater is a cultural centerpiece, attracting theater enthusiasts from far and wide.
Popular destination for winter sports.
Elektrostal’s proximity to ski resorts and winter sport facilities makes it a favorite destination for skiing, snowboarding, and other winter activities.
Promotes environmental sustainability.
Elektrostal prioritizes environmental protection and sustainability, implementing initiatives to reduce pollution and preserve natural resources.
Home to renowned educational institutions.
Elektrostal is known for its prestigious schools and universities, offering a wide range of academic programs to students.
Committed to cultural preservation.
The city values its cultural heritage and takes active steps to preserve and promote traditional customs, crafts, and arts.
Hosts an annual International Film Festival.
The Elektrostal International Film Festival attracts filmmakers and cinema enthusiasts from around the world, showcasing a diverse range of films.
Encourages entrepreneurship and innovation.
Elektrostal supports aspiring entrepreneurs and fosters a culture of innovation, providing opportunities for startups and business development.
Offers a range of housing options.
Elektrostal provides diverse housing options, including apartments, houses, and residential complexes, catering to different lifestyles and budgets.
Home to notable sports teams.
Elektrostal is proud of its sports legacy, with several successful sports teams competing at regional and national levels.
Boasts a vibrant nightlife scene.
Residents and visitors can enjoy a lively nightlife in Elektrostal, with numerous bars, clubs, and entertainment venues.
Promotes cultural exchange and international relations.
Elektrostal actively engages in international partnerships, cultural exchanges, and diplomatic collaborations to foster global connections.
Surrounded by beautiful nature reserves.
Nearby nature reserves, such as the Barybino Forest and Luchinskoye Lake, offer opportunities for nature enthusiasts to explore and appreciate the region’s biodiversity.
Commemorates historical events.
The city pays tribute to significant historical events through memorials, monuments, and exhibitions, ensuring the preservation of collective memory.
Promotes sports and youth development.
Elektrostal invests in sports infrastructure and programs to encourage youth participation, health, and physical fitness.
Hosts annual cultural and artistic festivals.
Throughout the year, Elektrostal celebrates its cultural diversity through festivals dedicated to music, dance, art, and theater.
Provides a picturesque landscape for photography enthusiasts.
The city’s scenic beauty, architectural landmarks, and natural surroundings make it a paradise for photographers.
Connects to Moscow via a direct train line.
The convenient train connection between Elektrostal and Moscow makes commuting between the two cities effortless.
A city with a bright future.
Elektrostal continues to grow and develop, aiming to become a model city in terms of infrastructure, sustainability, and quality of life for its residents.
In conclusion, Elektrostal is a fascinating city with a rich history and a vibrant present. From its origins as a center of steel production to its modern-day status as a hub for education and industry, Elektrostal has plenty to offer both residents and visitors. With its beautiful parks, cultural attractions, and proximity to Moscow, there is no shortage of things to see and do in this dynamic city. Whether you’re interested in exploring its historical landmarks, enjoying outdoor activities, or immersing yourself in the local culture, Elektrostal has something for everyone. So, next time you find yourself in the Moscow region, don’t miss the opportunity to discover the hidden gems of Elektrostal.
Q: What is the population of Elektrostal?
A: As of the latest data, the population of Elektrostal is approximately XXXX.
Q: How far is Elektrostal from Moscow?
A: Elektrostal is located approximately XX kilometers away from Moscow.
Q: Are there any famous landmarks in Elektrostal?
A: Yes, Elektrostal is home to several notable landmarks, including XXXX and XXXX.
Q: What industries are prominent in Elektrostal?
A: Elektrostal is known for its steel production industry and is also a center for engineering and manufacturing.
Q: Are there any universities or educational institutions in Elektrostal?
A: Yes, Elektrostal is home to XXXX University and several other educational institutions.
Q: What are some popular outdoor activities in Elektrostal?
A: Elektrostal offers several outdoor activities, such as hiking, cycling, and picnicking in its beautiful parks.
Q: Is Elektrostal well-connected in terms of transportation?
A: Yes, Elektrostal has good transportation links, including trains and buses, making it easily accessible from nearby cities.
Q: Are there any annual events or festivals in Elektrostal?
A: Yes, Elektrostal hosts various events and festivals throughout the year, including XXXX and XXXX.
Elektrostal's fascinating history, vibrant culture, and promising future make it a city worth exploring. For more captivating facts about cities around the world, discover the unique characteristics that define each city . Uncover the hidden gems of Moscow Oblast through our in-depth look at Kolomna. Lastly, dive into the rich industrial heritage of Teesside, a thriving industrial center with its own story to tell.
Was this page helpful?
Our commitment to delivering trustworthy and engaging content is at the heart of what we do. Each fact on our site is contributed by real users like you, bringing a wealth of diverse insights and information. To ensure the highest standards of accuracy and reliability, our dedicated editors meticulously review each submission. This process guarantees that the facts we share are not only fascinating but also credible. Trust in our commitment to quality and authenticity as you explore and learn with us.
Share this Fact:

IMAGES
VIDEO
COMMENTS
KCNK2. Potassium channel subfamily K member 2, also known as TREK-1, is a protein that in humans is encoded by the KCNK2 gene. [5] [6] [7] This gene encodes K 2P 2.1, a lipid-gated ion channel belonging to the two-pore-domain background potassium channel protein family. This type of potassium channel is formed by two homodimers that create a ...
TREK-1 is the most studied background K 2P channel. Its main role is to control cell excitability and maintain the membrane potential below the threshold of depolarization. TREK-1 is multi-regulated by a variety of physical and chemical stimuli which makes it a very promising and challenging target in the treatment of several pathologies. It is ...
TREK-1 is the most studied background K 2P channel. Its main role is to control cell excitability and maintain the membrane potential below the threshold of depolarization. TREK-1 is multi-regulated by a variety of physical and chemical stimuli which makes it a very promising and challengi …
TREK-1 is a two-pore-domain background potassium channel expressed throughout the central nervous system. It is opened by polyunsaturated fatty acids and lysophospholipids. It is inhibited by neurotransmitters that produce an increase in intracellular cAMP and by those that activate the Gq protein pathway. TREK-1 is also activated by volatile ...
The TREK-1 channel is a temperature-sensitive, osmosensitive and mechano-gated K+ channel with a regulation by Gs and Gq coupled receptors. This paper demonstrates that TREK-1 qualifies as one of the molecular sensors involved in pain perception. TREK-1 is highly expressed in small sensory neurons, is present in both peptidergic and ...
TREK-1 activation also decreased IL-6, IP-10, and CCL-2 secretion from primary AECs. Mechanistically, ML335 and BL1249 induced TREK-1 currents in AECs, counteracted HO-induced cell depolarization ...
The TREK-1 channel belongs to the TREK subfamily of two-pore domains channels that are activated by stretch and polyunsaturated fatty acids and inactivated by Protein Kinase A phosphorylation. The activation of this potassium channel must induce a hyperpolarization of the resting membrane potential and a shortening of the action potential ...
TREK-1 and TRAAK channels at nodes of Ranvier of myelinated afferent nerves are required for high-speed saltatory conduction and for sensory behavioral responses. A) Left, experimental setting. Right, traces illustrate APs recorded at NRs of shScramble and shTREK-1 groups. Arrow indicates stimulation marks.
TREK‐1 is a two‐pore‐domain background potassium channel expressed throughout the central nervous system. It is opened by polyunsaturated fatty acids and lysophospholipids. It is inhibited by neurotransmitters that produce an increase in intracellular cAMP and by those that activate the Gq protein pathway.
TREK-1 open reading frame was supplemented, at its 5′-end, with a sequence coding for a biotin acceptor domain (BAD) and a sequence coding for the thrombin protease cleavage site. The cleavage site was flanked by Gly-Leu-Asn-Gly-Gly toward BAD and Gly-Gly-Ser-Ala-Gly toward TREK-1. The fused gene was cloned in pYeDP60 expression plasmid (a ...
TREK-1 and TRAAK channels at NRs are shown in the present study to be essential for saltatory conduction at a high speed along myelinated afferent nerves. This important role of the leak K + channels may be due to the enhanced nodal membrane input conductance or the decreased nodal membrane input resistance, a result of the clustering of the ...
TREK-1 is multi-regulated by a variety of physical and chemical stimuli which makes it a very promising and challenging target in the treatment of several pathologies. It is mainly expressed in the brain but also in heart, smooth muscle cells, endocrine pancreas, and prostate.
218 Reviews / Write a Review. $499.99 $599.99. Model 1042794. Retailer prices may vary. FX 1 is a hybrid bike with a lightweight aluminum frame and quality parts that perform wherever you like to ride. It's perfect for anyone looking to get out more, ride as a family, do a bit of exercise, or commute to work on a versatile bike backed by a ...
The TREK‐1 channel is a temperature‐sensitive, osmosensitive and mechano‐gated K + channel with a regulation by Gs and Gq coupled receptors. This paper demonstrates that TREK‐1 qualifies as one of the molecular sensors involved in pain perception. TREK‐1 is highly expressed in small sensory neurons, is present in both peptidergic and ...
Trek started in a small Wisconsin barn in 1976, but our founders always saw something bigger. Decades later, we're on a mission to make our world a better place to live and ride. We build only products we love, provide incredible hospitality to our customers, and change the world by getting more people on bikes. ...
Star Trek: The Next Generation Season 1 is available to watch on Paramount Plus. Owned by Paramount World, Paramount Plus is an American subscription-based streaming service.
The sight of Captain Michael Burnham and Lieutenant Sylvia Tilly running the Race of the Mother Compeer together in Star Trek: Discovery season 5, episode 6, "Whistlespeak", calls back to when Specialist Burnham helps Cadet Tilly prepare for Starfleet's Command Training program in Star Trek: Discovery season 1. When Burnham and Tilly first meet as roommates in Star Trek: Discovery season 1 ...
TREK-1 is a two-pore-domain background potassium channel expressed throughout the central nervous system. It is opened by polyunsaturated fatty acids and lysophospholipids. It is inhibited by neurotransmitters that produce an increase in intracellular cAMP and by those that activate the Gq protein p …
On the "Star Trek" recap chat show "The Ready Room," hosted by Wil Wheaton, Kurtzman was asked about the future of "Star Trek," and the exec was, perhaps predictably, diplomatic. He couldn't very ...
TREK-1, a two-pore-domain K + channel, is highly expressed in the central nervous system. Although aberrant expression of TREK-1 is implicated in cognitive impairment, the cellular and functional mechanism underlying this channelopathy is poorly understood. Here we examined TREK-1 contribu …
INTRODUCTION. TREK-1 (Tandem of pore domain in a weak inwardly rectifying K + channel (Twik)-related K + channels) is one of the K + channels in the two-pore-domain potassium channel (K2P) family. TREK-1 shows high expression across the brain from the cortex, hippocampus to the spinal cord [].As a leak type K + channel, TREK-1 plays a role in setting the neuronal resting potential and ...
Elektrostal, city, Moscow oblast (province), western Russia.It lies 36 miles (58 km) east of Moscow city. The name, meaning "electric steel," derives from the high-quality-steel industry established there soon after the October Revolution in 1917. During World War II, parts of the heavy-machine-building industry were relocated there from Ukraine, and Elektrostal is now a centre for the ...
After a 20-year career on screen, including franchises Star Trek, ... Movies // 1 day ago 'Despicable Me 4' trailer shows creation of Mega Minions. May 7 (UPI) -- "Despicable Me 4," a new film in ...
Since the original Star Trek series first premiered on television in 1966, the Star Trek story and fictional sci-fi universe has evolved into several iterations, including Star Trek: The Next ...
The Moscow Metro is the third most intensive subway system in the world after Tokyo and Seoul subways. The first line was opened on May 15, 1935.
TREK-1 is also expressed in the heart, but its role in myocardial ischemia-reperfusion (IR)-induced injury has not been examined. In the current study, we used a TREK-1 knockout (KO) mouse model to show that TREK-1 has a critical role in the cardiac I/R-induced injury and during remodeling after myocardial infarction (MI).
Shatner hasn't starred in a 'Star Trek' story since 2006. William Shatner expressed interest in returning as Captain Kirk, desiring a substantial role, not just a cameo. Shatner considers de-aging ...
The 9th radio centre of Moscow was a high power shortwave and medium wave broadcasting facility at Elektrostal near Moscow.Its broadcasting frequency was 873 kHz with a transmission power of up to 1200 kilowatts. It was also used as radio jammer of "unwanted" stations.
40 Facts About Elektrostal. Elektrostal is a vibrant city located in the Moscow Oblast region of Russia. With a rich history, stunning architecture, and a thriving community, Elektrostal is a city that has much to offer. Whether you are a history buff, nature enthusiast, or simply curious about different cultures, Elektrostal is sure to ...