- Random article
- Teaching guide
- Privacy & cookies
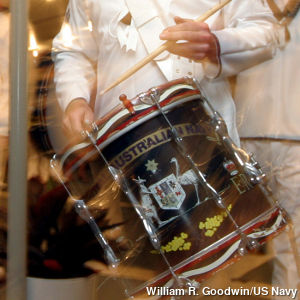
by Chris Woodford . Last updated: July 23, 2023.
Photo: Sound is energy we hear made by things that vibrate. Photo by William R. Goodwin courtesy of US Navy and Wikimedia Commons .

What is sound?
Photo: Sensing with sound: Light doesn't travel well through ocean water: over half the light falling on the sea surface is absorbed within the first meter of water; 100m down and only 1 percent of the surface light remains. That's largely why mighty creatures of the deep rely on sound for communication and navigation. Whales, famously, "talk" to one another across entire ocean basins, while dolphins use sound, like bats, for echolocation. Photo by Bill Thompson courtesy of US Fish and Wildlife Service .
Robert Boyle's classic experiment
Artwork: Robert Boyle's famous experiment with an alarm clock.
How sound travels
Artwork: Sound waves and ocean waves compared. Top: Sound waves are longitudinal waves: the air moves back and forth along the same line as the wave travels, making alternate patterns of compressions and rarefactions. Bottom: Ocean waves are transverse waves: the water moves back and forth at right angles to the line in which the wave travels.
The science of sound waves
Picture: Reflected sound is extremely useful for "seeing" underwater where light doesn't really travel—that's the basic idea behind sonar. Here's a side-scan sonar (reflected sound) image of a World War II boat wrecked on the seabed. Photo courtesy of U.S. National Oceanographic and Atmospheric Administration, US Navy, and Wikimedia Commons .
Whispering galleries and amphitheaters
Photos by Carol M. Highsmith: 1) The Capitol in Washington, DC has a whispering gallery inside its dome. Photo credit: The George F. Landegger Collection of District of Columbia Photographs in Carol M. Highsmith's America, Library of Congress , Prints and Photographs Division. 2) It's easy to hear people talking in the curved memorial amphitheater building at Arlington National Cemetery, Arlington, Virginia. Photo credit: Photographs in the Carol M. Highsmith Archive, Library of Congress , Prints and Photographs Division.
Measuring waves
Understanding amplitude and frequency, why instruments sound different, the speed of sound.
Photo: Breaking through the sound barrier creates a sonic boom. The mist you can see, which is called a condensation cloud, isn't necessarily caused by an aircraft flying supersonic: it can occur at lower speeds too. It happens because moist air condenses due to the shock waves created by the plane. You might expect the plane to compress the air as it slices through. But the shock waves it generates alternately expand and contract the air, producing both compressions and rarefactions. The rarefactions cause very low pressure and it's these that make moisture in the air condense, producing the cloud you see here. Photo by John Gay courtesy of US Navy and Wikimedia Commons .
Why does sound go faster in some things than in others?
Chart: Generally, sound travels faster in solids (right) than in liquids (middle) or gases (left)... but there are exceptions!
How to measure the speed of sound
Sound in practice, if you liked this article..., find out more, on this website.
- Electric guitars
- Speech synthesis
- Synthesizers
On other sites
- Explore Sound : A comprehensive educational site from the Acoustical Society of America, with activities for students of all ages.
- Sound Waves : A great collection of interactive science lessons from the University of Salford, which explains what sound waves are and the different ways in which they behave.
Educational books for younger readers
- Sound (Science in a Flash) by Georgia Amson-Bradshaw. Franklin Watts/Hachette, 2020. Simple facts, experiments, and quizzes fill this book; the visually exciting design will appeal to reluctant readers. Also for ages 7–9.
- Sound by Angela Royston. Raintree, 2017. A basic introduction to sound and musical sounds, including simple activities. Ages 7–9.
- Experimenting with Sound Science Projects by Robert Gardner. Enslow Publishers, 2013. A comprehensive 120-page introduction, running through the science of sound in some detail, with plenty of hands-on projects and activities (including welcome coverage of how to run controlled experiments using the scientific method). Ages 9–12.
- Cool Science: Experiments with Sound and Hearing by Chris Woodford. Gareth Stevens Inc, 2010. One of my own books, this is a short introduction to sound through practical activities, for ages 9–12.
- Adventures in Sound with Max Axiom, Super Scientist by Emily Sohn. Capstone, 2007. The original, graphic novel (comic book) format should appeal to reluctant readers. Ages 8–10.
Popular science
- The Sound Book: The Science of the Sonic Wonders of the World by Trevor Cox. W. W. Norton, 2014. An entertaining tour through everyday sound science.
Academic books
- Master Handbook of Acoustics by F. Alton Everest and Ken Pohlmann. McGraw-Hill Education, 2015. A comprehensive reference for undergraduates and sound-design professionals.
- The Science of Sound by Thomas D. Rossing, Paul A. Wheeler, and F. Richard Moore. Pearson, 2013. One of the most popular general undergraduate texts.
Text copyright © Chris Woodford 2009, 2021. All rights reserved. Full copyright notice and terms of use .
Rate this page
Tell your friends, cite this page, more to explore on our website....
- Get the book
- Send feedback
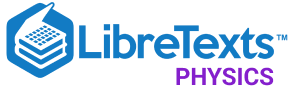
- school Campus Bookshelves
- menu_book Bookshelves
- perm_media Learning Objects
- login Login
- how_to_reg Request Instructor Account
- hub Instructor Commons
- Download Page (PDF)
- Download Full Book (PDF)
- Periodic Table
- Physics Constants
- Scientific Calculator
- Reference & Cite
- Tools expand_more
- Readability
selected template will load here
This action is not available.
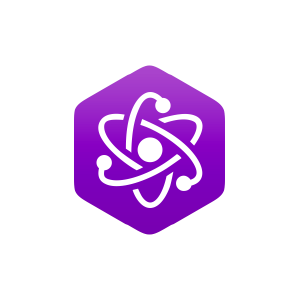
17.3: Speed of Sound
- Last updated
- Save as PDF
- Page ID 4077
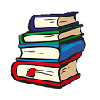
Learning Objectives
- Explain the relationship between wavelength and frequency of sound
- Determine the speed of sound in different media
- Derive the equation for the speed of sound in air
- Determine the speed of sound in air for a given temperature
Sound, like all waves, travels at a certain speed and has the properties of frequency and wavelength. You can observe direct evidence of the speed of sound while watching a fireworks display (Figure \(\PageIndex{1}\)). You see the flash of an explosion well before you hear its sound and possibly feel the pressure wave, implying both that sound travels at a finite speed and that it is much slower than light.
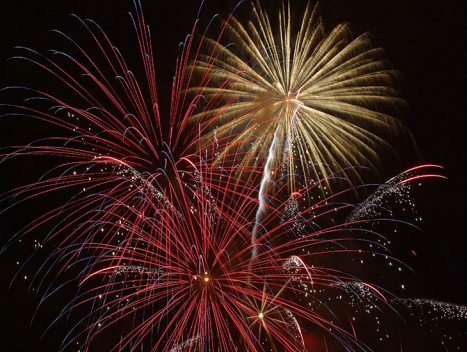
The difference between the speed of light and the speed of sound can also be experienced during an electrical storm. The flash of lighting is often seen before the clap of thunder. You may have heard that if you count the number of seconds between the flash and the sound, you can estimate the distance to the source. Every five seconds converts to about one mile. The velocity of any wave is related to its frequency and wavelength by
\[v = f \lambda, \label{17.3}\]
where \(v\) is the speed of the wave, \(f\) is its frequency, and \(\lambda\) is its wavelength. Recall from Waves that the wavelength is the length of the wave as measured between sequential identical points. For example, for a surface water wave or sinusoidal wave on a string, the wavelength can be measured between any two convenient sequential points with the same height and slope, such as between two sequential crests or two sequential troughs. Similarly, the wavelength of a sound wave is the distance between sequential identical parts of a wave—for example, between sequential compressions (Figure \(\PageIndex{2}\)). The frequency is the same as that of the source and is the number of waves that pass a point per unit time.
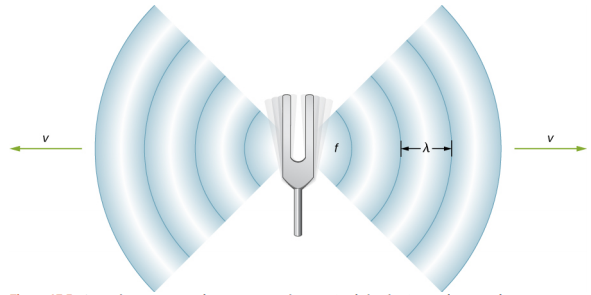
Speed of Sound in Various Media
Table \(\PageIndex{1}\) shows that the speed of sound varies greatly in different media. The speed of sound in a medium depends on how quickly vibrational energy can be transferred through the medium. For this reason, the derivation of the speed of sound in a medium depends on the medium and on the state of the medium. In general, the equation for the speed of a mechanical wave in a medium depends on the square root of the restoring force, or the elastic property, divided by the inertial property,
\[v = \sqrt{\frac{\text{elastic property}}{\text{inertial property}}} \ldotp\]
Also, sound waves satisfy the wave equation derived in Waves ,
\[\frac{\partial^{2} y (x,t)}{\partial x^{2}} = \frac{1}{v^{2}} \frac{\partial^{2} y (x,t)}{\partial t^{2}} \ldotp\]
Recall from Waves that the speed of a wave on a string is equal to \(v = \sqrt{\frac{F_{T}}{\mu}}\), where the restoring force is the tension in the string F T and the linear density \(\mu\) is the inertial property. In a fluid, the speed of sound depends on the bulk modulus and the density,
\[v = \sqrt{\frac{B}{\rho}} \ldotp \label{17.4}\]
The speed of sound in a solid the depends on the Young’s modulus of the medium and the density,
\[v = \sqrt{\frac{Y}{\rho}} \ldotp \label{17.5}\]
In an ideal gas (see The Kinetic Theory of Gases ), the equation for the speed of sound is
\[v = \sqrt{\frac{\gamma RT_{K}}{M}}, \label{17.6}\]
where \(\gamma\) is the adiabatic index, R = 8.31 J/mol • K is the gas constant, T K is the absolute temperature in kelvins, and M is the molecular mass. In general, the more rigid (or less compressible) the medium, the faster the speed of sound. This observation is analogous to the fact that the frequency of simple harmonic motion is directly proportional to the stiffness of the oscillating object as measured by k, the spring constant. The greater the density of a medium, the slower the speed of sound. This observation is analogous to the fact that the frequency of a simple harmonic motion is inversely proportional to m, the mass of the oscillating object. The speed of sound in air is low, because air is easily compressible. Because liquids and solids are relatively rigid and very difficult to compress, the speed of sound in such media is generally greater than in gases.
Because the speed of sound depends on the density of the material, and the density depends on the temperature, there is a relationship between the temperature in a given medium and the speed of sound in the medium. For air at sea level, the speed of sound is given by
\[v = 331\; m/s \sqrt{1 + \frac{T_{C}}{273 °C}} = 331\; m/s \sqrt{\frac{T_{K}}{273\; K}} \label{17.7}\]
where the temperature in the first equation (denoted as T C ) is in degrees Celsius and the temperature in the second equation (denoted as T K ) is in kelvins. The speed of sound in gases is related to the average speed of particles in the gas,
\[v_{rms} = \sqrt{\frac{3k_{B}T}{m}}.\]
where \(k_B\) is the Boltzmann constant (1.38 x 10 −23 J/K) and m is the mass of each (identical) particle in the gas. Note that v refers to the speed of the coherent propagation of a disturbance (the wave), whereas \(v_{rms}\) describes the speeds of particles in random directions. Thus, it is reasonable that the speed of sound in air and other gases should depend on the square root of temperature. While not negligible, this is not a strong dependence. At 0°C , the speed of sound is 331 m/s, whereas at 20.0 °C, it is 343 m/s, less than a 4% increase. Figure \(\PageIndex{3}\) shows how a bat uses the speed of sound to sense distances.
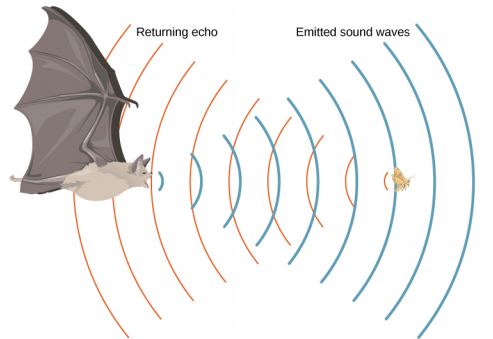
Derivation of the Speed of Sound in Air
As stated earlier, the speed of sound in a medium depends on the medium and the state of the medium. The derivation of the equation for the speed of sound in air starts with the mass flow rate and continuity equation discussed in Fluid Mechanics . Consider fluid flow through a pipe with cross-sectional area \(A\) (Figure \(\PageIndex{4}\)). The mass in a small volume of length \(x\) of the pipe is equal to the density times the volume, or
\[m = \rho V = \rho Ax.\]
The mass flow rate is
\[\frac{dm}{dt} = \frac{d}{dt} (\rho V) = \frac{d}{dt} (\rho Ax) = \rho A \frac{dx}{dt} = \rho Av \ldotp\]
The continuity equation from Fluid Mechanics states that the mass flow rate into a volume has to equal the mass flow rate out of the volume,
\[\rho_{in} A_{in}v_{in} = \rho_{out} A_{out}v_{out}.\]
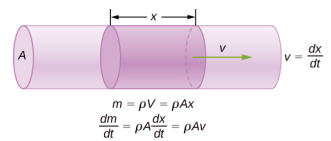
Now consider a sound wave moving through a parcel of air. A parcel of air is a small volume of air with imaginary boundaries (Figure \(\PageIndex{5}\)). The density, temperature, and velocity on one side of the volume of the fluid are given as \(\rho\), T, v, and on the other side are \(\rho\) + d\(\rho\), \(T + dT\), \(v + dv\).
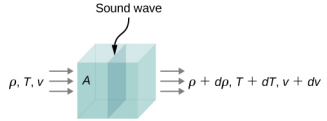
The continuity equation states that the mass flow rate entering the volume is equal to the mass flow rate leaving the volume, so
\[\rho Av = (\rho + d \rho)A(v + dv) \ldotp\]
This equation can be simplified, noting that the area cancels and considering that the multiplication of two infinitesimals is approximately equal to zero: d\(\rho\)(dv) ≈ 0,
\[\begin{split} \rho v & = (\rho + d \rho)(v + dv) \\ & = \rho v + \rho (dv) + (d \rho)v + (d \rho)(dv) \\ 0 & = \rho (dv) + (d \rho) v \\ \rho\; dv & = -v\; d \rho \ldotp \end{split}\]
The net force on the volume of fluid (Figure \(\PageIndex{6}\)) equals the sum of the forces on the left face and the right face:
\[\begin{split} F_{net} & = p\; dy\; dz - (p + dp)\; dy\; dz \ & = p\; dy\; dz\; - p\; dy\; dz - dp\; dy\; dz \\ & = -dp\; dy\; dz \\ ma & = -dp\; dy\; dz \ldotp \end{split}\]
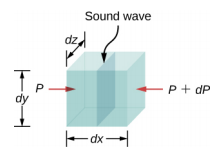
Figure \(\PageIndex{6}\):
The acceleration is the force divided by the mass and the mass is equal to the density times the volume, m = \(\rho\)V = \(\rho\) dx dy dz. We have
\[\begin{split} ma & = -dp\; dy\; dz \\ a & = - \frac{dp\; dy\; dz}{m} = - \frac{dp\; dy\; dz}{\rho\; dx\; dy\; dz} = - \frac{dp}{\rho\; dx} \\ \frac{dv}{dt} & = - \frac{dp}{\rho\; dx} \\ dv & = - \frac{dp}{\rho dx} dt = - \frac{dp}{\rho} \frac{1}{v} \\ \rho v\; dv & = -dp \ldotp \end{split}\]
From the continuity equation \(\rho\) dv = −vd\(\rho\), we obtain
\[\begin{split} \rho v\; dv & = -dp \\ (-v\; d \rho)v & = -dp \\ v & = \sqrt{\frac{dp}{d \rho}} \ldotp \end{split}\]
Consider a sound wave moving through air. During the process of compression and expansion of the gas, no heat is added or removed from the system. A process where heat is not added or removed from the system is known as an adiabatic system. Adiabatic processes are covered in detail in The First Law of Thermodynamics , but for now it is sufficient to say that for an adiabatic process, \(pV^{\gamma} = \text{constant}\), where \(p\) is the pressure, \(V\) is the volume, and gamma (\(\gamma\)) is a constant that depends on the gas. For air, \(\gamma\) = 1.40. The density equals the number of moles times the molar mass divided by the volume, so the volume is equal to V = \(\frac{nM}{\rho}\). The number of moles and the molar mass are constant and can be absorbed into the constant p \(\left(\dfrac{1}{\rho}\right)^{\gamma}\) = constant. Taking the natural logarithm of both sides yields ln p − \(\gamma\) ln \(\rho\) = constant. Differentiating with respect to the density, the equation becomes
\[\begin{split} \ln p - \gamma \ln \rho & = constant \\ \frac{d}{d \rho} (\ln p - \gamma \ln \rho) & = \frac{d}{d \rho} (constant) \\ \frac{1}{p} \frac{dp}{d \rho} - \frac{\gamma}{\rho} & = 0 \\ \frac{dp}{d \rho} & = \frac{\gamma p}{\rho} \ldotp \end{split}\]
If the air can be considered an ideal gas, we can use the ideal gas law:
\[\begin{split} pV & = nRT = \frac{m}{M} RT \\ p & = \frac{m}{V} \frac{RT}{M} = \rho \frac{RT}{M} \ldotp \end{split}\]
Here M is the molar mass of air:
\[\frac{dp}{d \rho} = \frac{\gamma p}{\rho} = \frac{\gamma \left(\rho \frac{RT}{M}\right)}{\rho} = \frac{\gamma RT}{M} \ldotp\]
Since the speed of sound is equal to v = \(\sqrt{\frac{dp}{d \rho}}\), the speed is equal to
\[v = \sqrt{\frac{\gamma RT}{M}} \ldotp\]
Note that the velocity is faster at higher temperatures and slower for heavier gases. For air, \(\gamma\) = 1.4, M = 0.02897 kg/mol, and R = 8.31 J/mol • K. If the temperature is T C = 20 °C (T = 293 K), the speed of sound is v = 343 m/s. The equation for the speed of sound in air v = \(\sqrt{\frac{\gamma RT}{M}}\) can be simplified to give the equation for the speed of sound in air as a function of absolute temperature:
\[\begin{split} v & = \sqrt{\frac{\gamma RT}{M}} \\ & = \sqrt{\frac{\gamma RT}{M} \left(\dfrac{273\; K}{273\; K}\right)} = \sqrt{\frac{(273\; K) \gamma R}{M}} \sqrt{\frac{T}{273\; K}} \\ & \approx 331\; m/s \sqrt{\frac{T}{273\; K}} \ldotp \end{split}\]
One of the more important properties of sound is that its speed is nearly independent of the frequency. This independence is certainly true in open air for sounds in the audible range. If this independence were not true, you would certainly notice it for music played by a marching band in a football stadium, for example. Suppose that high-frequency sounds traveled faster—then the farther you were from the band, the more the sound from the low-pitch instruments would lag that from the high-pitch ones. But the music from all instruments arrives in cadence independent of distance, so all frequencies must travel at nearly the same speed. Recall that
\[v = f \lambda \ldotp\]
In a given medium under fixed conditions, \(v\) is constant, so there is a relationship between \(f\) and \(\lambda\); the higher the frequency, the smaller the wavelength (Figure \(\PageIndex{7}\)).
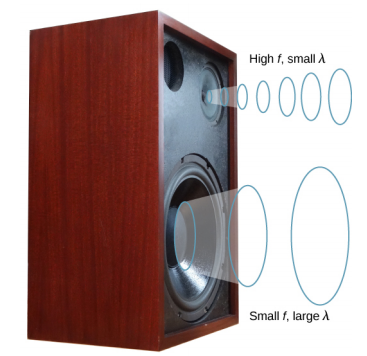
Example \(\PageIndex{1}\): Calculating Wavelengths
Calculate the wavelengths of sounds at the extremes of the audible range, 20 and 20,000 Hz, in 30.0 °C air. (Assume that the frequency values are accurate to two significant figures.)
To find wavelength from frequency, we can use \(v = f \lambda\).
- Identify knowns. The value for \(v\) is given by \[v = 331\; m/s \sqrt{\frac{T}{273\; K}} \ldotp \nonumber\]
- Convert the temperature into kelvins and then enter the temperature into the equation \[v = 331\; m/s \sqrt{\frac{303\; K}{273\; K}} = 348.7\; m/s \ldotp \nonumber\]
- Solve the relationship between speed and wavelength for \(\lambda\): $$\lambda = \frac{v}{f} \ldotp \nonumber$$
- Enter the speed and the minimum frequency to give the maximum wavelength: \[\lambda_{max} = \frac{348.7\; m/s}{20\; Hz} = 17\; m \ldotp \nonumber\]
- Enter the speed and the maximum frequency to give the minimum wavelength: \[\lambda_{min} = \frac{348.7\; m/s}{20,000\; Hz} = 0.017\; m = 1.7\; cm \ldotp \nonumber\]
Significance
Because the product of \(f\) multiplied by \(\lambda\) equals a constant, the smaller \(f\) is, the larger \(\lambda\) must be, and vice versa.
The speed of sound can change when sound travels from one medium to another, but the frequency usually remains the same. This is similar to the frequency of a wave on a string being equal to the frequency of the force oscillating the string. If \(v\) changes and \(f\) remains the same, then the wavelength \(\lambda\) must change. That is, because \(v = f \lambda\), the higher the speed of a sound, the greater its wavelength for a given frequency.
Exercise \(\PageIndex{1}\)
Imagine you observe two firework shells explode. You hear the explosion of one as soon as you see it. However, you see the other shell for several milliseconds before you hear the explosion. Explain why this is so.
Although sound waves in a fluid are longitudinal, sound waves in a solid travel both as longitudinal waves and transverse waves. Seismic waves, which are essentially sound waves in Earth’s crust produced by earthquakes, are an interesting example of how the speed of sound depends on the rigidity of the medium. Earthquakes produce both longitudinal and transverse waves, and these travel at different speeds. The bulk modulus of granite is greater than its shear modulus. For that reason, the speed of longitudinal or pressure waves (P-waves) in earthquakes in granite is significantly higher than the speed of transverse or shear waves (S-waves). Both types of earthquake waves travel slower in less rigid material, such as sediments. P-waves have speeds of 4 to 7 km/s, and S-waves range in speed from 2 to 5 km/s, both being faster in more rigid material. The P-wave gets progressively farther ahead of the S-wave as they travel through Earth’s crust. The time between the P- and S-waves is routinely used to determine the distance to their source, the epicenter of the earthquake. Because S-waves do not pass through the liquid core, two shadow regions are produced (Figure \(\PageIndex{8}\)).
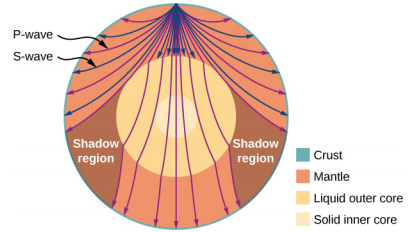
As sound waves move away from a speaker, or away from the epicenter of an earthquake, their power per unit area decreases. This is why the sound is very loud near a speaker and becomes less loud as you move away from the speaker. This also explains why there can be an extreme amount of damage at the epicenter of an earthquake but only tremors are felt in areas far from the epicenter. The power per unit area is known as the intensity, and in the next section, we will discuss how the intensity depends on the distance from the source.
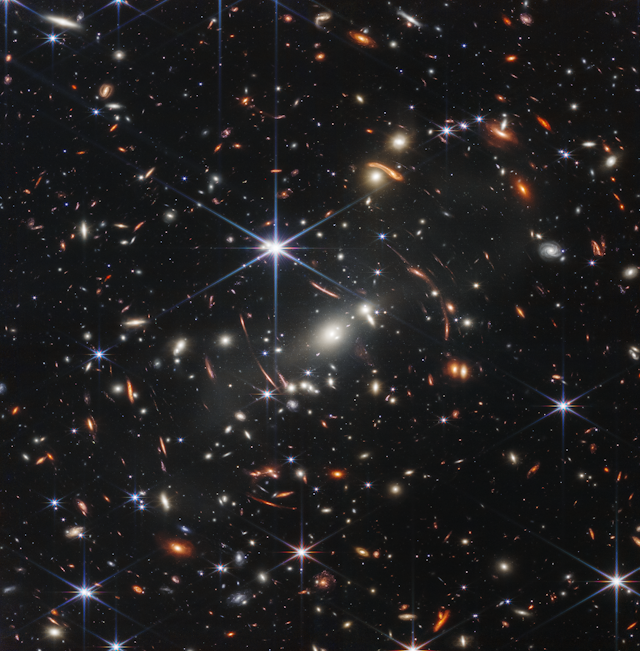
Why isn’t there any sound in space? An astronomer explains why in space no one can hear you scream
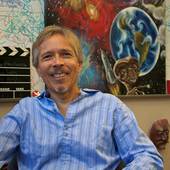
University Distinguished Professor of Astronomy, University of Arizona
Disclosure statement
Chris Impey receives funding from the National Science Foundation.
University of Arizona provides funding as a member of The Conversation US.
View all partners
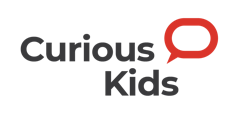
Curious Kids is a series for children of all ages. If you have a question you’d like an expert to answer, send it to [email protected] .
How far can sound travel through space, since it’s so empty? Is there an echo in space? – Jasmine, age 14, Everson, Washington
In space, no one can hear you scream.
You may have heard this saying. It’s the tagline from the famous 1979 science fiction movie “ Alien .” It’s a scary thought, but is it true? The simple answer is yes, no one can hear you scream in space because there is no sound or echo in space.
I’m a professor of astronomy , which means I study space and how it works. Space is silent – for the most part.
How sound works
To understand why there’s no sound in space, first consider how sound works. Sound is a wave of energy that moves through a solid, a liquid or a gas.
Sound is a compression wave . The energy created when your vocal cords vibrate slightly compresses the air in your throat, and the compressed energy travels outward.
A good analogy for sound is a Slinky toy . If you stretch out a Slinky and push hard on one end, a compression wave travels down the Slinky.
When you talk, your vocal cords vibrate. They jostle air molecules in your throat above your vocal cords, which in turn jostle or bump into their neighbors, causing a sound to come out of your mouth.
Sound moves through air the same way it moves through your throat. Air molecules near your mouth bump into their neighbors, which in turn bump into their neighbors, and the sound moves through the air. The sound wave travels quickly , about 760 miles per hour (1,223 kilometers per hour), which is faster than a commercial jet.
Space is a vacuum
So what about in space?
Space is a vacuum, which means it contains almost no matter. The word vacuum comes from the Latin word for empty .
Sound is carried by atoms and molecules. In space, with no atoms or molecules to carry a sound wave, there’s no sound. There’s nothing to get in sound’s way out in space, but there’s nothing to carry it, so it doesn’t travel at all. No sound also means no echo. An echo happens when a sound wave hits a hard, flat surface and bounces back in the direction it came from.
By the way, if you were caught in space outside your spacecraft with no spacesuit, the fact that no one could hear your cry for help is the least of your problems. Any air you still had in your lungs would expand because it was at higher pressure than the vacuum outside. Your lungs would rupture. In a mere 10 to 15 seconds , you’d be unconscious due to a lack of oxygen.
Sound in the solar system
Scientists have wondered how human voices would sound on our nearest neighboring planets, Venus and Mars. This experiment is hypothetical because Mars is usually below freezing , and its atmosphere is thin, unbreathable carbon dioxide . Venus is even worse – its air is hot enough to melt lead, with a thick carbon dioxide atmosphere.
On Mars, your voice would sound tinny and hollow, like the sound of a piccolo . On Venus , the pitch of your voice would be much deeper, like the sound of a booming bass guitar. The reason is the thickness of the atmosphere. On Mars the thin air creates a high-pitched sound, and on Venus the thick air creates a low-pitched sound. The team that worked this out simulated other solar system sounds , like a waterfall on Saturn’s moon Titan.
Deep space sounds
While space is a good enough vacuum that normal sound can’t travel through it, it’s actually not a perfect vacuum, and it does have some particles floating through it.
Beyond the Earth and its atmosphere, there are five particles in a typical cubic centimeter – the volume of a sugar cube – that are mostly hydrogen atoms. By contrast, the air you are breathing is 10 billion billion (10 19) times more dense. The density goes down with distance from the Sun, and in the space between stars there are 0.1 particles per cubic centimeter. In vast voids between galaxies , it is a million times lower still – fantastically empty.
The voids of space are kept very hot by radiation from stars. The very spread-out matter found there is in a physical state called a plasma .
A plasma is a gas in which electrons are separated from protons. In a plasma, the physics of sound waves get complicated . Waves travel much faster in this low-density medium, and their wavelength is much longer.
In 2022, NASA released a spectacular example of sound in space . It used X-ray data to make an audible recording that represents the way a massive black hole stirs up plasma in the Perseus galaxy cluster, 250 million light years from Earth. The black hole itself emits no sound, but the diffuse plasma around it carries very long wavelength sound waves.
The natural sound is far too low a frequency for the human ear to hear, 57 octaves below middle C, which is the middle note on a piano and in the middle of the range of sound people can hear. But after raising the frequency to the audible range, the result is chilling – it’s the sound of a black hole growling in deep space.
Hello, curious kids! Do you have a question you’d like an expert to answer? Ask an adult to send your question to [email protected] . Please tell us your name, age and the city where you live.
And since curiosity has no age limit – adults, let us know what you’re wondering, too. We won’t be able to answer every question, but we will do our best.
- Astrophysics
- Curious Kids
- Curious Kids US
- Vocal cords

Biocloud Project Manager - Australian Biocommons

Director, Defence and Security

Opportunities with the new CIEHF

School of Social Sciences – Public Policy and International Relations opportunities

Deputy Editor - Technology
17.1 Sound Waves
Learning objectives.
By the end of this section, you will be able to:
- Explain the difference between sound and hearing
- Describe sound as a wave
- List the equations used to model sound waves
- Describe compression and rarefactions as they relate to sound
The physical phenomenon of sound is a disturbance of matter that is transmitted from its source outward. Hearing is the perception of sound, just as seeing is the perception of visible light. On the atomic scale, sound is a disturbance of atoms that is far more ordered than their thermal motions. In many instances, sound is a periodic wave, and the atoms undergo simple harmonic motion. Thus, sound waves can induce oscillations and resonance effects ( Figure 17.2 ).
Interactive
This video shows waves on the surface of a wine glass, being driven by sound waves from a speaker. As the frequency of the sound wave approaches the resonant frequency of the wine glass, the amplitude and frequency of the waves on the wine glass increase. When the resonant frequency is reached, the glass shatters.
A speaker produces a sound wave by oscillating a cone, causing vibrations of air molecules. In Figure 17.3 , a speaker vibrates at a constant frequency and amplitude, producing vibrations in the surrounding air molecules. As the speaker oscillates back and forth, it transfers energy to the air, mostly as thermal energy. But a small part of the speaker’s energy goes into compressing and expanding the surrounding air, creating slightly higher and lower local pressures. These compressions (high-pressure regions) and rarefactions (low-pressure regions) move out as longitudinal pressure waves having the same frequency as the speaker—they are the disturbance that is a sound wave. (Sound waves in air and most fluids are longitudinal, because fluids have almost no shear strength. In solids, sound waves can be both transverse and longitudinal.)
Figure 17.3 (a) shows the compressions and rarefactions, and also shows a graph of gauge pressure versus distance from a speaker. As the speaker moves in the positive x -direction, it pushes air molecules, displacing them from their equilibrium positions. As the speaker moves in the negative x -direction, the air molecules move back toward their equilibrium positions due to a restoring force. The air molecules oscillate in simple harmonic motion about their equilibrium positions, as shown in part (b). Note that sound waves in air are longitudinal, and in the figure, the wave propagates in the positive x -direction and the molecules oscillate parallel to the direction in which the wave propagates.
Models Describing Sound
Sound can be modeled as a pressure wave by considering the change in pressure from average pressure,
This equation is similar to the periodic wave equations seen in Waves , where Δ P Δ P is the change in pressure, Δ P max Δ P max is the maximum change in pressure, k = 2 π λ k = 2 π λ is the wave number, ω = 2 π T = 2 π f ω = 2 π T = 2 π f is the angular frequency, and ϕ ϕ is the initial phase. The wave speed can be determined from v = ω k = λ T . v = ω k = λ T . Sound waves can also be modeled in terms of the displacement of the air molecules. The displacement of the air molecules can be modeled using a cosine function:
In this equation, s is the displacement and s max s max is the maximum displacement.
Not shown in the figure is the amplitude of a sound wave as it decreases with distance from its source, because the energy of the wave is spread over a larger and larger area. The intensity decreases as it moves away from the speaker, as discussed in Waves . The energy is also absorbed by objects and converted into thermal energy by the viscosity of the air. In addition, during each compression, a little heat transfers to the air; during each rarefaction, even less heat transfers from the air, and these heat transfers reduce the organized disturbance into random thermal motions. Whether the heat transfer from compression to rarefaction is significant depends on how far apart they are—that is, it depends on wavelength. Wavelength, frequency, amplitude, and speed of propagation are important characteristics for sound, as they are for all waves.
As an Amazon Associate we earn from qualifying purchases.
This book may not be used in the training of large language models or otherwise be ingested into large language models or generative AI offerings without OpenStax's permission.
Want to cite, share, or modify this book? This book uses the Creative Commons Attribution License and you must attribute OpenStax.
Access for free at https://openstax.org/books/university-physics-volume-1/pages/1-introduction
- Authors: William Moebs, Samuel J. Ling, Jeff Sanny
- Publisher/website: OpenStax
- Book title: University Physics Volume 1
- Publication date: Sep 19, 2016
- Location: Houston, Texas
- Book URL: https://openstax.org/books/university-physics-volume-1/pages/1-introduction
- Section URL: https://openstax.org/books/university-physics-volume-1/pages/17-1-sound-waves
© Jan 19, 2024 OpenStax. Textbook content produced by OpenStax is licensed under a Creative Commons Attribution License . The OpenStax name, OpenStax logo, OpenStax book covers, OpenStax CNX name, and OpenStax CNX logo are not subject to the Creative Commons license and may not be reproduced without the prior and express written consent of Rice University.
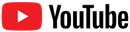
- TPC and eLearning
- Read Watch Interact
- What's NEW at TPC?
- Practice Review Test
- Teacher-Tools
- Subscription Selection
- Seat Calculator
- Ad Free Account
- Edit Profile Settings
- Classes (Version 2)
- Student Progress Edit
- Task Properties
- Export Student Progress
- Task, Activities, and Scores
- Metric Conversions Questions
- Metric System Questions
- Metric Estimation Questions
- Significant Digits Questions
- Proportional Reasoning
- Acceleration
- Distance-Displacement
- Dots and Graphs
- Graph That Motion
- Match That Graph
- Name That Motion
- Motion Diagrams
- Pos'n Time Graphs Numerical
- Pos'n Time Graphs Conceptual
- Up And Down - Questions
- Balanced vs. Unbalanced Forces
- Change of State
- Force and Motion
- Mass and Weight
- Match That Free-Body Diagram
- Net Force (and Acceleration) Ranking Tasks
- Newton's Second Law
- Normal Force Card Sort
- Recognizing Forces
- Air Resistance and Skydiving
- Solve It! with Newton's Second Law
- Which One Doesn't Belong?
- Component Addition Questions
- Head-to-Tail Vector Addition
- Projectile Mathematics
- Trajectory - Angle Launched Projectiles
- Trajectory - Horizontally Launched Projectiles
- Vector Addition
- Vector Direction
- Which One Doesn't Belong? Projectile Motion
- Forces in 2-Dimensions
- Being Impulsive About Momentum
- Explosions - Law Breakers
- Hit and Stick Collisions - Law Breakers
- Case Studies: Impulse and Force
- Impulse-Momentum Change Table
- Keeping Track of Momentum - Hit and Stick
- Keeping Track of Momentum - Hit and Bounce
- What's Up (and Down) with KE and PE?
- Energy Conservation Questions
- Energy Dissipation Questions
- Energy Ranking Tasks
- LOL Charts (a.k.a., Energy Bar Charts)
- Match That Bar Chart
- Words and Charts Questions
- Name That Energy
- Stepping Up with PE and KE Questions
- Case Studies - Circular Motion
- Circular Logic
- Forces and Free-Body Diagrams in Circular Motion
- Gravitational Field Strength
- Universal Gravitation
- Angular Position and Displacement
- Linear and Angular Velocity
- Angular Acceleration
- Rotational Inertia
- Balanced vs. Unbalanced Torques
- Getting a Handle on Torque
- Torque-ing About Rotation
- Properties of Matter
- Fluid Pressure
- Buoyant Force
- Sinking, Floating, and Hanging
- Pascal's Principle
- Flow Velocity
- Bernoulli's Principle
- Balloon Interactions
- Charge and Charging
- Charge Interactions
- Charging by Induction
- Conductors and Insulators
- Coulombs Law
- Electric Field
- Electric Field Intensity
- Polarization
- Case Studies: Electric Power
- Know Your Potential
- Light Bulb Anatomy
- I = ∆V/R Equations as a Guide to Thinking
- Parallel Circuits - ∆V = I•R Calculations
- Resistance Ranking Tasks
- Series Circuits - ∆V = I•R Calculations
- Series vs. Parallel Circuits
- Equivalent Resistance
- Period and Frequency of a Pendulum
- Pendulum Motion: Velocity and Force
- Energy of a Pendulum
- Period and Frequency of a Mass on a Spring
- Horizontal Springs: Velocity and Force
- Vertical Springs: Velocity and Force
- Energy of a Mass on a Spring
- Decibel Scale
- Frequency and Period
- Closed-End Air Columns
- Name That Harmonic: Strings
- Rocking the Boat
- Wave Basics
- Matching Pairs: Wave Characteristics
- Wave Interference
- Waves - Case Studies
- Color Addition and Subtraction
- Color Filters
- If This, Then That: Color Subtraction
- Light Intensity
- Color Pigments
- Converging Lenses
- Curved Mirror Images
- Law of Reflection
- Refraction and Lenses
- Total Internal Reflection
- Who Can See Who?
- Formulas and Atom Counting
- Atomic Models
- Bond Polarity
- Entropy Questions
- Cell Voltage Questions
- Heat of Formation Questions
- Reduction Potential Questions
- Oxidation States Questions
- Measuring the Quantity of Heat
- Hess's Law
- Oxidation-Reduction Questions
- Galvanic Cells Questions
- Thermal Stoichiometry
- Molecular Polarity
- Quantum Mechanics
- Balancing Chemical Equations
- Bronsted-Lowry Model of Acids and Bases
- Classification of Matter
- Collision Model of Reaction Rates
- Density Ranking Tasks
- Dissociation Reactions
- Complete Electron Configurations
- Elemental Measures
- Enthalpy Change Questions
- Equilibrium Concept
- Equilibrium Constant Expression
- Equilibrium Calculations - Questions
- Equilibrium ICE Table
- Ionic Bonding
- Lewis Electron Dot Structures
- Limiting Reactants
- Line Spectra Questions
- Measurement and Numbers
- Metals, Nonmetals, and Metalloids
- Metric Estimations
- Metric System
- Molarity Ranking Tasks
- Mole Conversions
- Name That Element
- Names to Formulas
- Names to Formulas 2
- Nuclear Decay
- Particles, Words, and Formulas
- Periodic Trends
- Precipitation Reactions and Net Ionic Equations
- Pressure Concepts
- Pressure-Temperature Gas Law
- Pressure-Volume Gas Law
- Chemical Reaction Types
- Significant Digits and Measurement
- States Of Matter Exercise
- Stoichiometry Law Breakers
- Stoichiometry - Math Relationships
- Subatomic Particles
- Spontaneity and Driving Forces
- Gibbs Free Energy
- Volume-Temperature Gas Law
- Acid-Base Properties
- Energy and Chemical Reactions
- Chemical and Physical Properties
- Valence Shell Electron Pair Repulsion Theory
- Writing Balanced Chemical Equations
- Mission CG1
- Mission CG10
- Mission CG2
- Mission CG3
- Mission CG4
- Mission CG5
- Mission CG6
- Mission CG7
- Mission CG8
- Mission CG9
- Mission EC1
- Mission EC10
- Mission EC11
- Mission EC12
- Mission EC2
- Mission EC3
- Mission EC4
- Mission EC5
- Mission EC6
- Mission EC7
- Mission EC8
- Mission EC9
- Mission RL1
- Mission RL2
- Mission RL3
- Mission RL4
- Mission RL5
- Mission RL6
- Mission KG7
- Mission RL8
- Mission KG9
- Mission RL10
- Mission RL11
- Mission RM1
- Mission RM2
- Mission RM3
- Mission RM4
- Mission RM5
- Mission RM6
- Mission RM8
- Mission RM10
- Mission LC1
- Mission RM11
- Mission LC2
- Mission LC3
- Mission LC4
- Mission LC5
- Mission LC6
- Mission LC8
- Mission SM1
- Mission SM2
- Mission SM3
- Mission SM4
- Mission SM5
- Mission SM6
- Mission SM8
- Mission SM10
- Mission KG10
- Mission SM11
- Mission KG2
- Mission KG3
- Mission KG4
- Mission KG5
- Mission KG6
- Mission KG8
- Mission KG11
- Mission F2D1
- Mission F2D2
- Mission F2D3
- Mission F2D4
- Mission F2D5
- Mission F2D6
- Mission KC1
- Mission KC2
- Mission KC3
- Mission KC4
- Mission KC5
- Mission KC6
- Mission KC7
- Mission KC8
- Mission AAA
- Mission SM9
- Mission LC7
- Mission LC9
- Mission NL1
- Mission NL2
- Mission NL3
- Mission NL4
- Mission NL5
- Mission NL6
- Mission NL7
- Mission NL8
- Mission NL9
- Mission NL10
- Mission NL11
- Mission NL12
- Mission MC1
- Mission MC10
- Mission MC2
- Mission MC3
- Mission MC4
- Mission MC5
- Mission MC6
- Mission MC7
- Mission MC8
- Mission MC9
- Mission RM7
- Mission RM9
- Mission RL7
- Mission RL9
- Mission SM7
- Mission SE1
- Mission SE10
- Mission SE11
- Mission SE12
- Mission SE2
- Mission SE3
- Mission SE4
- Mission SE5
- Mission SE6
- Mission SE7
- Mission SE8
- Mission SE9
- Mission VP1
- Mission VP10
- Mission VP2
- Mission VP3
- Mission VP4
- Mission VP5
- Mission VP6
- Mission VP7
- Mission VP8
- Mission VP9
- Mission WM1
- Mission WM2
- Mission WM3
- Mission WM4
- Mission WM5
- Mission WM6
- Mission WM7
- Mission WM8
- Mission WE1
- Mission WE10
- Mission WE2
- Mission WE3
- Mission WE4
- Mission WE5
- Mission WE6
- Mission WE7
- Mission WE8
- Mission WE9
- Vector Walk Interactive
- Name That Motion Interactive
- Kinematic Graphing 1 Concept Checker
- Kinematic Graphing 2 Concept Checker
- Graph That Motion Interactive
- Two Stage Rocket Interactive
- Rocket Sled Concept Checker
- Force Concept Checker
- Free-Body Diagrams Concept Checker
- Free-Body Diagrams The Sequel Concept Checker
- Skydiving Concept Checker
- Elevator Ride Concept Checker
- Vector Addition Concept Checker
- Vector Walk in Two Dimensions Interactive
- Name That Vector Interactive
- River Boat Simulator Concept Checker
- Projectile Simulator 2 Concept Checker
- Projectile Simulator 3 Concept Checker
- Hit the Target Interactive
- Turd the Target 1 Interactive
- Turd the Target 2 Interactive
- Balance It Interactive
- Go For The Gold Interactive
- Egg Drop Concept Checker
- Fish Catch Concept Checker
- Exploding Carts Concept Checker
- Collision Carts - Inelastic Collisions Concept Checker
- Its All Uphill Concept Checker
- Stopping Distance Concept Checker
- Chart That Motion Interactive
- Roller Coaster Model Concept Checker
- Uniform Circular Motion Concept Checker
- Horizontal Circle Simulation Concept Checker
- Vertical Circle Simulation Concept Checker
- Race Track Concept Checker
- Gravitational Fields Concept Checker
- Orbital Motion Concept Checker
- Angular Acceleration Concept Checker
- Balance Beam Concept Checker
- Torque Balancer Concept Checker
- Aluminum Can Polarization Concept Checker
- Charging Concept Checker
- Name That Charge Simulation
- Coulomb's Law Concept Checker
- Electric Field Lines Concept Checker
- Put the Charge in the Goal Concept Checker
- Circuit Builder Concept Checker (Series Circuits)
- Circuit Builder Concept Checker (Parallel Circuits)
- Circuit Builder Concept Checker (∆V-I-R)
- Circuit Builder Concept Checker (Voltage Drop)
- Equivalent Resistance Interactive
- Pendulum Motion Simulation Concept Checker
- Mass on a Spring Simulation Concept Checker
- Particle Wave Simulation Concept Checker
- Boundary Behavior Simulation Concept Checker
- Slinky Wave Simulator Concept Checker
- Simple Wave Simulator Concept Checker
- Wave Addition Simulation Concept Checker
- Standing Wave Maker Simulation Concept Checker
- Color Addition Concept Checker
- Painting With CMY Concept Checker
- Stage Lighting Concept Checker
- Filtering Away Concept Checker
- InterferencePatterns Concept Checker
- Young's Experiment Interactive
- Plane Mirror Images Interactive
- Who Can See Who Concept Checker
- Optics Bench (Mirrors) Concept Checker
- Name That Image (Mirrors) Interactive
- Refraction Concept Checker
- Total Internal Reflection Concept Checker
- Optics Bench (Lenses) Concept Checker
- Kinematics Preview
- Velocity Time Graphs Preview
- Moving Cart on an Inclined Plane Preview
- Stopping Distance Preview
- Cart, Bricks, and Bands Preview
- Fan Cart Study Preview
- Friction Preview
- Coffee Filter Lab Preview
- Friction, Speed, and Stopping Distance Preview
- Up and Down Preview
- Projectile Range Preview
- Ballistics Preview
- Juggling Preview
- Marshmallow Launcher Preview
- Air Bag Safety Preview
- Colliding Carts Preview
- Collisions Preview
- Engineering Safer Helmets Preview
- Push the Plow Preview
- Its All Uphill Preview
- Energy on an Incline Preview
- Modeling Roller Coasters Preview
- Hot Wheels Stopping Distance Preview
- Ball Bat Collision Preview
- Energy in Fields Preview
- Weightlessness Training Preview
- Roller Coaster Loops Preview
- Universal Gravitation Preview
- Keplers Laws Preview
- Kepler's Third Law Preview
- Charge Interactions Preview
- Sticky Tape Experiments Preview
- Wire Gauge Preview
- Voltage, Current, and Resistance Preview
- Light Bulb Resistance Preview
- Series and Parallel Circuits Preview
- Thermal Equilibrium Preview
- Linear Expansion Preview
- Heating Curves Preview
- Electricity and Magnetism - Part 1 Preview
- Electricity and Magnetism - Part 2 Preview
- Vibrating Mass on a Spring Preview
- Period of a Pendulum Preview
- Wave Speed Preview
- Slinky-Experiments Preview
- Standing Waves in a Rope Preview
- Sound as a Pressure Wave Preview
- DeciBel Scale Preview
- DeciBels, Phons, and Sones Preview
- Sound of Music Preview
- Shedding Light on Light Bulbs Preview
- Models of Light Preview
- Electromagnetic Radiation Preview
- Electromagnetic Spectrum Preview
- EM Wave Communication Preview
- Digitized Data Preview
- Light Intensity Preview
- Concave Mirrors Preview
- Object Image Relations Preview
- Snells Law Preview
- Reflection vs. Transmission Preview
- Magnification Lab Preview
- Reactivity Preview
- Ions and the Periodic Table Preview
- Periodic Trends Preview
- Reaction Rates Preview
- Ammonia Factory Preview
- Stoichiometry Preview
- Gaining Teacher Access
- Tasks and Classes
- Tasks - Classic
- Subscription
- Subscription Locator
- 1-D Kinematics
- Newton's Laws
- Vectors - Motion and Forces in Two Dimensions
- Momentum and Its Conservation
- Work and Energy
- Circular Motion and Satellite Motion
- Thermal Physics
- Static Electricity
- Electric Circuits
- Vibrations and Waves
- Sound Waves and Music
- Light and Color
- Reflection and Mirrors
- About the Physics Interactives
- Task Tracker
- Usage Policy
- Newtons Laws
- Vectors and Projectiles
- Forces in 2D
- Momentum and Collisions
- Circular and Satellite Motion
- Balance and Rotation
- Electromagnetism
- Waves and Sound
- Forces in Two Dimensions
- Work, Energy, and Power
- Circular Motion and Gravitation
- Sound Waves
- 1-Dimensional Kinematics
- Circular, Satellite, and Rotational Motion
- Einstein's Theory of Special Relativity
- Waves, Sound and Light
- QuickTime Movies
- About the Concept Builders
- Pricing For Schools
- Directions for Version 2
- Measurement and Units
- Relationships and Graphs
- Rotation and Balance
- Vibrational Motion
- Reflection and Refraction
- Teacher Accounts
- Task Tracker Directions
- Kinematic Concepts
- Kinematic Graphing
- Wave Motion
- Sound and Music
- About CalcPad
- 1D Kinematics
- Vectors and Forces in 2D
- Simple Harmonic Motion
- Rotational Kinematics
- Rotation and Torque
- Rotational Dynamics
- Electric Fields, Potential, and Capacitance
- Transient RC Circuits
- Light Waves
- Units and Measurement
- Stoichiometry
- Molarity and Solutions
- Thermal Chemistry
- Acids and Bases
- Kinetics and Equilibrium
- Solution Equilibria
- Oxidation-Reduction
- Nuclear Chemistry
- NGSS Alignments
- 1D-Kinematics
- Projectiles
- Circular Motion
- Magnetism and Electromagnetism
- Graphing Practice
- About the ACT
- ACT Preparation
- For Teachers
- Other Resources
- Newton's Laws of Motion
- Work and Energy Packet
- Static Electricity Review
- Solutions Guide
- Solutions Guide Digital Download
- Motion in One Dimension
- Work, Energy and Power
- Frequently Asked Questions
- Purchasing the Download
- Purchasing the CD
- Purchasing the Digital Download
- About the NGSS Corner
- NGSS Search
- Force and Motion DCIs - High School
- Energy DCIs - High School
- Wave Applications DCIs - High School
- Force and Motion PEs - High School
- Energy PEs - High School
- Wave Applications PEs - High School
- Crosscutting Concepts
- The Practices
- Physics Topics
- NGSS Corner: Activity List
- NGSS Corner: Infographics
- About the Toolkits
- Position-Velocity-Acceleration
- Position-Time Graphs
- Velocity-Time Graphs
- Newton's First Law
- Newton's Second Law
- Newton's Third Law
- Terminal Velocity
- Projectile Motion
- Forces in 2 Dimensions
- Impulse and Momentum Change
- Momentum Conservation
- Work-Energy Fundamentals
- Work-Energy Relationship
- Roller Coaster Physics
- Satellite Motion
- Electric Fields
- Circuit Concepts
- Series Circuits
- Parallel Circuits
- Describing-Waves
- Wave Behavior Toolkit
- Standing Wave Patterns
- Resonating Air Columns
- Wave Model of Light
- Plane Mirrors
- Curved Mirrors
- Teacher Guide
- Using Lab Notebooks
- Current Electricity
- Light Waves and Color
- Reflection and Ray Model of Light
- Refraction and Ray Model of Light
- Classes (Legacy Version)
- Teacher Resources
- Subscriptions

- Newton's Laws
- Einstein's Theory of Special Relativity
- About Concept Checkers
- School Pricing
- Newton's Laws of Motion
- Newton's First Law
- Newton's Third Law

Sound as a Longitudinal Wave
- Sound is a Mechanical Wave
- Sound is a Longitudinal Wave
- Sound is a Pressure Wave
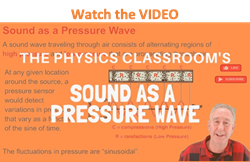
Sound waves in air (and any fluid medium) are longitudinal waves because particles of the medium through which the sound is transported vibrate parallel to the direction that the sound wave moves. A vibrating string can create longitudinal waves as depicted in the animation below. As the vibrating string moves in the forward direction, it begins to push upon surrounding air molecules, moving them to the right towards their nearest neighbor. This causes the air molecules to the right of the string to be compressed into a small region of space. As the vibrating string moves in the reverse direction (leftward), it lowers the pressure of the air immediately to its right, thus causing air molecules to move back leftward. The lower pressure to the right of the string causes air molecules in that region immediately to the right of the string to expand into a large region of space. The back and forth vibration of the string causes individual air molecules (or a layer of air molecules) in the region immediately to the right of the string to continually vibrate back and forth horizontally. The molecules move rightward as the string moves rightward and then leftward as the string moves leftward. These back and forth vibrations are imparted to adjacent neighbors by particle-to-particle interaction. Other surrounding particles begin to move rightward and leftward, thus sending a wave to the right. Since air molecules (the particles of the medium) are moving in a direction that is parallel to the direction that the wave moves, the sound wave is referred to as a longitudinal wave. The result of such longitudinal vibrations is the creation of compressions and rarefactions within the air.
Regardless of the source of the sound wave - whether it is a vibrating string or the vibrating tines of a tuning fork - sound waves traveling through air are longitudinal waves. And the essential characteristic of a longitudinal wave that distinguishes it from other types of waves is that the particles of the medium move in a direction parallel to the direction of energy transport.
We Would Like to Suggest ...
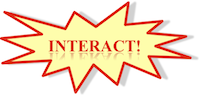
- Pitch and Frequency
Sciencing_Icons_Science SCIENCE
Sciencing_icons_biology biology, sciencing_icons_cells cells, sciencing_icons_molecular molecular, sciencing_icons_microorganisms microorganisms, sciencing_icons_genetics genetics, sciencing_icons_human body human body, sciencing_icons_ecology ecology, sciencing_icons_chemistry chemistry, sciencing_icons_atomic & molecular structure atomic & molecular structure, sciencing_icons_bonds bonds, sciencing_icons_reactions reactions, sciencing_icons_stoichiometry stoichiometry, sciencing_icons_solutions solutions, sciencing_icons_acids & bases acids & bases, sciencing_icons_thermodynamics thermodynamics, sciencing_icons_organic chemistry organic chemistry, sciencing_icons_physics physics, sciencing_icons_fundamentals-physics fundamentals, sciencing_icons_electronics electronics, sciencing_icons_waves waves, sciencing_icons_energy energy, sciencing_icons_fluid fluid, sciencing_icons_astronomy astronomy, sciencing_icons_geology geology, sciencing_icons_fundamentals-geology fundamentals, sciencing_icons_minerals & rocks minerals & rocks, sciencing_icons_earth scructure earth structure, sciencing_icons_fossils fossils, sciencing_icons_natural disasters natural disasters, sciencing_icons_nature nature, sciencing_icons_ecosystems ecosystems, sciencing_icons_environment environment, sciencing_icons_insects insects, sciencing_icons_plants & mushrooms plants & mushrooms, sciencing_icons_animals animals, sciencing_icons_math math, sciencing_icons_arithmetic arithmetic, sciencing_icons_addition & subtraction addition & subtraction, sciencing_icons_multiplication & division multiplication & division, sciencing_icons_decimals decimals, sciencing_icons_fractions fractions, sciencing_icons_conversions conversions, sciencing_icons_algebra algebra, sciencing_icons_working with units working with units, sciencing_icons_equations & expressions equations & expressions, sciencing_icons_ratios & proportions ratios & proportions, sciencing_icons_inequalities inequalities, sciencing_icons_exponents & logarithms exponents & logarithms, sciencing_icons_factorization factorization, sciencing_icons_functions functions, sciencing_icons_linear equations linear equations, sciencing_icons_graphs graphs, sciencing_icons_quadratics quadratics, sciencing_icons_polynomials polynomials, sciencing_icons_geometry geometry, sciencing_icons_fundamentals-geometry fundamentals, sciencing_icons_cartesian cartesian, sciencing_icons_circles circles, sciencing_icons_solids solids, sciencing_icons_trigonometry trigonometry, sciencing_icons_probability-statistics probability & statistics, sciencing_icons_mean-median-mode mean/median/mode, sciencing_icons_independent-dependent variables independent/dependent variables, sciencing_icons_deviation deviation, sciencing_icons_correlation correlation, sciencing_icons_sampling sampling, sciencing_icons_distributions distributions, sciencing_icons_probability probability, sciencing_icons_calculus calculus, sciencing_icons_differentiation-integration differentiation/integration, sciencing_icons_application application, sciencing_icons_projects projects, sciencing_icons_news news.
- Share Tweet Email Print
- Home ⋅
- Science ⋅
- Physics ⋅
- Sound & Light (Physics): How are They Different?
How Do Sound Waves Travel?
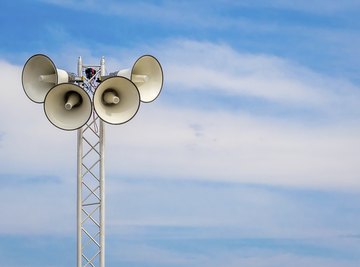
Sound: Definition, Types, Characteristics & Frequencies
In physics, a wave is a disturbance that travels through a medium such as air or water, and moves energy from one place to another. Sound waves, as the name implies, bear a form of energy that our biological sensory equipment -- i.e., our ears and brains -- recognize as noise, be it the pleasant sound of music or the grating cacophony of a jackhammer.
Basic Properties
Sound waves have several features in common with other waves. One is that they must have a substrate, or medium, in which to travel; some are more suitable than others. A second is that they must have a source -- say, the plucking of a guitar string or two hands clapping together. A third is that they transmit energy through direct particle-to-particle interaction, which means that they are a type of mechanical wave.
Sound waves can travel through any material, but not in a vacuum, which is why there is no sound in outer space. The speed of sound in air is about 330 m/s, meaning that it covers a mile in about five seconds. Sound actually travels at far quicker speeds in other media; for example, in biological tissues, it moves at 1,540 m/s.
Related Articles
How to calculate photons per second, properties of infrared light, infrared vs. visible light, differences between protozoa & protists, how is light transmitted, what is the formula for velocity of a wave, how does light travel, how to calculate hertz to joules, how does a transducer work, how does water affect sound, type of energy produced by photosynthesis, how to convert metric tons to cubic yards, how to convert nanometers to joules, the trophic levels of the barn owl, how to calculate frequency.
- The Physics Classroom: Sound Is a Mechanical Wave
- The Physics Hypertextbook: The Nature of Sound
About the Author
Michael Crystal earned a Bachelor of Science in biology at Case Western Reserve University, where he was a varsity distance runner, and is a USA Track and Field-certified coach. Formerly the editor of his running club's newsletter, he has been published in "Trail Runner Magazine" and "Men's Health." He is pursuing a medical degree.
Photo Credits
John Kelly/iStock/Getty Images
Find Your Next Great Science Fair Project! GO
We Have More Great Sciencing Articles!
Kinetic Energy: Definition, Formula, Types (w/ Examples)
Sound & light (physics): how are they different, waves (properties & characteristics): amplitude, period, frequency, wavelength.
How do sound waves work?
Sound waves are vibrations that can move us, hurt us, and maybe even heal us.
By Brian S. Hawkins | Updated Jun 1, 2023 2:00 PM EDT

We live our entire lives surrounded by them. They slam into us constantly at more than 700 miles per hour, sometimes hurting, sometimes soothing . They have the power to communicate ideas, evoke fond memories, start fights, entertain an audience, scare the heck out of us, or help us fall in love. They can trigger a range of emotions and they even cause physical damage. This reads like something out of science fiction , but what we’re talking about is very much real and already part of our day-to-day lives. They’re sound waves. So, what are sound waves and how do they work?
If you’re not in the industry of audio, you probably don’t think too much about the mechanics of sound. Sure, most people care about how sounds make them feel, but they aren’t as concerned with how the sound actually affects them. Understanding how sound works does have a number of practical applications , however, and you don’t have to be a physicist or engineer to explore this fascinating subject. Here’s a primer on the science of sound to help get you started.
What’s in a wave
When energy moves through a substance such as water or air, it makes a wave. There are two kinds of waves: longitudinal ones and transverse ones. Transverse waves, as NASA notes , are probably what most people think of when they picture waves—like the up-down ripples of a battle rope used to work out. Longitudinal waves are also known as compression waves, and that’s what sound waves are. There’s no perpendicular motion to these, rather, the wave moves in the same direction as the disturbance.
How sound waves work
Sound waves are a type of energy that’s released when an object vibrates. Those acoustic waves travel from their source through air or another medium, and when they come into contact with our eardrums, our brains translate the pressure waves into words, music, or signals we can understand. These pulses help you place where things are in your environment.
We can experience sound waves in ways that are more physical, not just physiological, too. If sound waves reach a microphone —whether it’s a plug-n-play USB livestream mic or a studio-quality microphone for vocals —it transforms them into electronic impulses that are turned back into sound by vibrating speakers . Whether listening at home or at a concert, we can feel the deep bass in our chest. Opera singers can use them to shatter glass. It’s even possible to see sound waves sent through a medium like sand, which leaves behind a kind of sonic footprint.
That shape is rolling peaks and valleys, the signature of a sine (aka sinusoid) wave. If the wave travels faster, those peaks and valleys form closer together. If it moves slower, they spread out. It’s not a poor analogy to think of them somewhat like waves in the ocean. It’s this movement that allows sound waves to do so many other things.
It’s all about frequency
When we talk about a sound wave’s speed, we’re referring to how fast these longitudinal waves move from peak to trough and back to peak. Up … and then down … and then up … and then down. The technical term is frequency , but many of us know it as pitch. We measure sound frequency in hertz (Hz), which represents cycles-per-second, with faster frequencies creating higher-pitched sounds. For instance, the A note right above Middle C on a piano is measured at 440 Hz—it travels up and down at 440 cycles per second. Middle C itself is 261.63 Hz—a lower pitch, vibrating at a slower frequency.
Understanding frequencies can be useful in many ways. You can precisely tune an instrument by analyzing the frequencies of its strings. Recording engineers use their understanding of frequency ranges to dial in equalization settings that help sculpt the sound of the music they’re mixing . Car designers work with frequencies—and materials that can block them—to help make engines quieter. And active noise cancellation uses artificial intelligence and algorithms to measure external frequencies and generate inverse waves to cancel environmental rumble and hum, allowing top-tier ANC headphones and earphones to isolate the wearer from the noise around them. The average frequency range of human hearing is 20 to 20,000 Hz.
What’s in a name?
The hertz measurement is named for the German physicist Heinrich Rudolf Hertz , who proved the existence of electromagnetic waves.
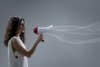
Getting amped
Amplitude equates to sound’s volume or intensity. Using our ocean analogy—because, hey, it works—amplitude describes the height of the waves.
We measure amplitude in decibels (dB). The dB scale is logarithmic, which means there’s a fixed ratio between measurement units. And what does that mean? Let’s say you have a dial on your guitar amp with evenly spaced steps on it numbered one through five. If the knob is following a logarithmic scale, the volume won’t increase evenly as you turn the dial from marker to marker. If the ratio is 4, let’s say, then turning the dial from the first to the second marker increases the sound by 4 dB. But going from the second to the third marker increases it by 16 dB. Turn the dial again and your amp becomes 64 dB louder. Turn it once more, and you’ll blast out a blistering 256 dB—more than loud enough to rupture your eardrums. But if you’re somehow still standing, you can turn that knob one more time to increase your volume to a brain-walloping 1,024 decibels. That’s almost 10 times louder than any rock concert you’ll ever encounter, and it will definitely get you kicked out of your rehearsal space. All of which is why real amps aren’t designed that way.
Twice as nice
We interpret a 10 dB increase in amplitude as a doubling of volume.
Parts of a sound wave
Timbre and envelope are two characteristics of sound waves that help determine why, say, two instruments can play the same chords but sound nothing alike.
Timbre is determined by the unique harmonics formed by the combination of notes in a chord. The A in an A chord is only its fundamental note—you also have overtones and undertones. The way these sound together helps keep a piano from sounding like a guitar, or an angry grizzly bear from sounding like a rumbling tractor engine.
[Related: Even plants pick up on good vibes ]
But we also rely on envelopes, which determine how a sound’s amplitude changes over time. A cello’s note might swell slowly to its maximum volume, then hold for a bit before gently fading out again. On the other hand, a slamming door delivers a quick, sharp, loud sound that cuts off almost instantly. Envelopes comprise four parts: Attack, Decay, Sustain, and Release. In fact, they’re more formally known as ADSR Envelopes.
- Attack: This is how quickly the sound achieves its maximum volume. A barking dog has a very short attack; a rising orchestra has a slower one.
- Decay: This describes how fast the sound settles into its sustained volume. When a guitar player plucks a string , the note starts off loudly but quickly settles into something quieter before fading out completely. The time it takes to hit that sustained volume is decay.
- Sustain: Sustain isn’t a measure of time; it’s a measure of amplitude, or volume. It’s how loud the plucked guitar note is after the initial attack but before it fades out.
- Release: This is the time it takes for the note to drift off to silence.
Speed of sound
Science fiction movies like it when spaceships explode with giant, rumbling, surround-sound booms . However, sound needs to travel through a medium so, despite Hollywood saying otherwise, you’d never hear an explosion in the vacuum of space.
Sound’s velocity , or the speed it travels at , differs depending on the density (and even temperature) of the medium it’s moving through—it’s faster in the air than water, for instance. Generally, sound moves at 1,127 feet per second, or 767.54 miles per hour. When jets break the sound barrier , they’re traveling faster than that. And knowing these numbers lets you estimate the distance of a lightning strike by counting the time between the flash and thunder’s boom—if you count to 10, it’s approximately 11,270 feet away, or about a quarter-mile. (Very roughly, of course.)
A stimulating experience
Anyone can benefit from understanding the fundamentals of sound and what sound waves are. Musicians and content creators with home recording set-ups and studio monitors obviously need a working knowledge of frequencies and amplitude. If you host a podcast, you’ll want as many tools as possible to ensure your voice sounds clear and rich, and this can include understanding the frequencies of your voice, what microphones are best suited to them , and how to set up your room to reflect or dampen the sounds you do or do not want. Having some foundational information is also useful when doing home-improvement projects— when treating a recording workstation , for instance, or just soundproofing a new enclosed deck. And who knows, maybe one day you’ll want to shatter glass. Having a better understanding of the physics of sound opens up wonderful new ways to explore and experience the world around us. Now, go out there and make some noise!
This post has been updated. It was originally published on July 27, 2021.

Brian is a documentary producer, director, and cameraman on feature films and docu-series, and has more than 20 years’ experience as a journalist. He enjoys covering pop-culture, tech, and the conflation of the two.
Like science, tech, and DIY projects?
Sign up to receive Popular Science's emails and get the highlights.
June 27, 2019
What Do You Hear Underwater?
A submerged science activity from Science Buddies
By Science Buddies & Sabine De Brabandere
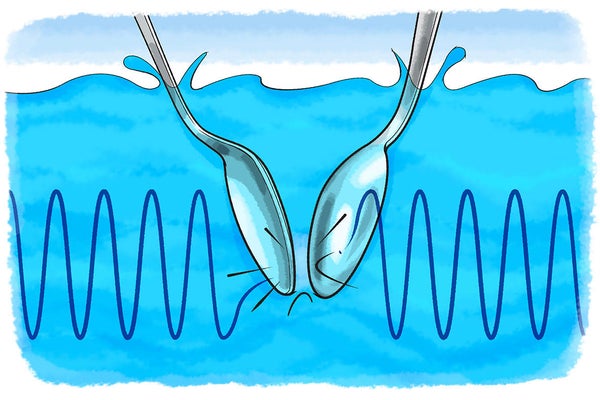
Make waves--underwater! Learn how sound travels differently in water than it does in the air.
George Retseck
Key Concepts Physics Sound Waves Biology
Introduction Have you ever listened to noises underwater? Sound travels differently in the water than it does in the air. To learn more, try making your own underwater noises—and listening carefully.
Background Sound is a wave created by vibrations. These vibrations create areas of more and less densely packed particles. So sound needs a medium to travel, such as air, water—or even solids.
On supporting science journalism
If you're enjoying this article, consider supporting our award-winning journalism by subscribing . By purchasing a subscription you are helping to ensure the future of impactful stories about the discoveries and ideas shaping our world today.
Sound waves travel faster in denser substances because neighboring particles will more easily bump into one another. Take water, for example. There are about 800 times more particles in a bottle of water than there are in the same bottle filled with air. Thus sound waves travel much faster in water than they do in air. In freshwater at room temperature, for example, sound travels about 4.3 times faster than it does in air at the same temperature.
Sound traveling through air soon becomes less loud as you get farther from the source. This is because the waves’ energy quickly gets lost along the way. Sound keeps its energy longer when traveling through water because the particles can carry the sound waves better. In the ocean, for example, the sound of a humpback whale can travel thousands of miles!
Underwater sound waves reaching us at a faster pace and keeping their intensity longer seem like they should make us perceive those sounds as louder when we are also underwater. The human ear, however, evolved to hear sound in the air and is not as useful when submerged in water. Our head itself is full of tissues that contain water and can transmit sound waves when we are underwater. When this happens, the vibrations bypass the eardrum, the part of the ear that evolved to pick up sound waves in the air.
Sound also interacts with boundaries between two different mediums, such as the surface of water. This boundary between water and air, for example, reflects almost all sounds back into the water. How will all these dynamics influence how we perceive underwater sounds? Try the activity to find out!
Bathtub or swimming pool (a very large bucket can work, too)
Two stainless steel utensils (for example, spoons or tongs)
Two plastic utensils
Small ball
Adult helper
An area that can get wet (if not performing the activity at a pool)
Floor cloth to cleanup spills (if not performing the activity at a pool)
Other materials to make underwater sounds (optional)
Access to a swimming pool (optional)
Internet access (optional)
Preparation
Fill the bathtub with lukewarm water—or head to the pool—and bring your helper and other materials.
Ask your helper to click one stainless steel utensil against another. Listen. How would you describe the sound?
In a moment, your helper will click one utensil against the other underwater . Do you think you will hear the same sound?
Ask your helper to click one utensil against the other underwater. Listen. Does the sound appear to be louder or softer? Is what you hear different in other ways, too?
Submerge one ear in the water. Ask your helper to click one utensil against the other underwater. Listen. How would you describe this sound?
Ask your helper to click one utensil against the other underwater soon after you submerge your head. Take a deep breath, close your eyes and submerge your head completely or as much as you feel comfortable doing. Listen while you hold your breath underwater (come up for air when you need to!). Does the sound appear to be louder or softer? Does it appear to be different in other ways?
Repeat this sequence but have your helper use two plastic utensils banging against each other instead.
Repeat the sequence again, but this time listen to a small ball being dropped into the water. Does the sound of a ball falling into the water change when you listen above or below water? Does your perception of this sound change? Why would this happen?
Switch roles. Have your helper listen while you make the sounds.
Discuss the findings you gathered. Do patterns appear? Can you conclude something about how humans perceive sounds when submerged in water?
Extra : Test with more types of sounds: soft as well as loud sounds, high- as well as low-pitched sounds. Can you find more patterns?
Extra: To investigate what picks up the sound wave when you are submerged, use your fingers to close your ears or use earbuds when submerging your head. How does the sound change when you close off your ear canal underwater? Does the same happen when you close off your ear canal when you are above water? If not, why would this be different?
Extra: Go to the swimming pool and listen to the sound of someone jumping into the water. Compare your perception of the sound when you are submerged with when your head is above the water. How does your perception change? Close your eyes. Can you tell where the person jumped into the water when submerged? Can you tell when you have your head above the water?
Extra: Research ocean sounds and how sounds caused by human activity impact aquatic animals.
Observations and Results Was the sound softer when it was created underwater and you listened above the water? Did it sound muffled when you had only your ear submerged? Was it fuller when you had your head submerged?
Sound travels faster in water compared with air because water particles are packed in more densely. Thus, the energy the sound waves carry is transported faster. This should make the sound appear louder. You probably perceived it as softer when you were not submerged, however, because the water surface is almost like a mirror for the sound you created. The sound most likely almost completely reflected back into the water as soon as it reached the surface.
When you submerged only your ear, the sound probably still appeared muffled. This happens because the human ear is not good at picking up sound in water—after all, it evolved to pick up sound in air.
When you submerged your head, the sound probably sounded fuller. That is because our head contains a lot of water, which allows the tissue to pick up underwater sound—without relying on the eardrum. It also explains why closing your ear canal makes almost no difference in the sound you pick up while you are underwater.
If you tried to detect where the sound came from when submerged, you probably had a hard time. Our brain uses the difference in loudness and timing of the sound detected by each ear as a clue to infer where the sound came from. Because sound travels faster underwater and because you pick up sound with your entire head when you are submerged, your brain loses the cues that normally help you determine where the sound is coming from.
More to Explore Discovery of Sound in the Sea , from the University of Rhode Island and the Inner Space Center Can You Hear Sounds in Outer Space? , from Science Buddies Talk through a String Telephone , from Scientific American Sound Localization , from Science Buddies Ears: Do Their Design, Size and Shape Matter? , from Scientific American STEM Activities for Kids , from Science Buddies
This activity brought to you in partnership with Science Buddies
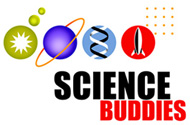
How far does sound travel in the ocean?
The distance that sound travels in the ocean varies greatly, depending primarily upon water temperature and pressure..

Water temperature and pressure determine how far sound travels in the ocean.
While sound moves at a much faster speed in the water than in air , the distance that sound waves travel is primarily dependent upon ocean temperature and pressure. While pressure continues to increase as ocean depth increases, the temperature of the ocean only decreases up to a certain point, after which it remains relatively stable. These factors have a curious effect on how (and how far) sound waves travel.
Imagine a whale is swimming through the ocean and calls out to its pod. The whale produces sound waves that move like ripples in the water. As the whale’s sound waves travel through the water, their speed decreases with increasing depth (as the temperature drops), causing the sound waves to refract downward . Once the sound waves reach the bottom of what is known as the thermocline layer, the speed of sound reaches its minimum. The thermocline is a region characterized by rapid change in temperature and pressure which occurs at different depths around the world. Below the thermocline "layer," the temperature remains constant, but pressure continues to increase. This causes the speed of sound to increase and makes the sound waves refract upward .
The area in the ocean where sound waves refract up and down is known as the "sound channel." The channeling of sound waves allows sound to travel thousands of miles without the signal losing considerable energy. In fact, hydrophones, or underwater microphones, if placed at the proper depth, can pick up whale songs and manmade noises from many kilometers away.
Search Our Facts
More information.
- Noise in the Ocean: A National Issue (National Marine Sanctuaries)
- Just how noisy is the ocean? Learn about a NOAA Effort to Monitor Underwater Sound
- Sound in the Sea Gallery
- Acoustic Monitoring
Last updated: 01/20/23 Author: NOAA How to cite this article

- Science Notes Posts
- Contact Science Notes
- Todd Helmenstine Biography
- Anne Helmenstine Biography
- Free Printable Periodic Tables (PDF and PNG)
- Periodic Table Wallpapers
- Interactive Periodic Table
- Periodic Table Posters
- How to Grow Crystals
- Chemistry Projects
- Fire and Flames Projects
- Holiday Science
- Chemistry Problems With Answers
- Physics Problems
- Unit Conversion Example Problems
- Chemistry Worksheets
- Biology Worksheets
- Periodic Table Worksheets
- Physical Science Worksheets
- Science Lab Worksheets
- My Amazon Books
Is There Sound in Space?

No, there is no sound in space. At least, not in the way we traditionally understand sound on Earth. The misconception exists largely due to popular culture. Movies and TV shows often depict space battles with roaring rockets and booming exploding stars, but in reality, space is eerily silent.
The reason for this silence lies in the nature of sound itself. Sound is a vibration that travels through a medium, like air or water. For sound waves to propagate, they need particles. Space is a near- perfect vacuum , meaning it has very few particles. Without a medium for these sound waves, there is no sound.
NASA’s “Space Sounds”: Understanding Sonification
Despite the silence of space, there are videos and recordings labeled as “sounds from space” released by NASA. These are not sounds in the traditional sense. Instead, they are products of a process called sonification.
Sonification is the conversion of data into sound. In the context of space, instruments on spacecraft record electromagnetic vibrations or particle interactions. These signals, which are not audible, get converted into sound waves that we can hear. When scientists represent data in an auditory format, it makes certain patterns and anomalies easier to detect.
For instance, the eerie “whistles” and “howls” from recordings of Jupiter or Saturn aren’t sounds that an astronaut could hear. Instead, they are sonifications of radio waves or other electromagnetic phenomena detected by spacecraft.
Gravitational Waves: A Type of Sound in Space
The groundbreaking discovery of gravitational waves adds a new layer to our understanding of “sounds” in space. Detected by the Laser Interferometer Gravitational-Wave Observatory (LIGO), these are ripples in spacetime caused by cataclysmic events, like the merging of two black holes.
Now, gravitational waves aren’t sounds in the traditional sense. They don’t propagate through air or water; they literally stretch and compress the fabric of the universe. However, much like the sonifications mentioned earlier, scientists often convert gravitational wave data into sound.
When scientists at LIGO do this, the results are astounding. The final moments of two black holes spiraling into one another can be “heard” as a chirp. In this context, these gravitational waves are akin to the universe’s symphony, a testament to the colossal events unfolding in the cosmos.
Sound in Space: Can You Hear Sound on the Moon?
Similar to the vastness of space, the Moon is also an environment where sound doesn’t propagate in the traditional manner. The Moon has an extremely thin atmosphere or exosphere, which consists of very few particles. Because of this near- vacuum condition, there’s no medium for sound waves to travel through on the Moon’s surface. So, if an astronaut shouts on the Moon without any equipment, the sound doesn’t travel. Another astronaut standing a distance can’t hear it.
How Astronauts Talk on the Moon
Given the lack of an effective medium for sound transmission on the Moon, you might wonder how astronauts communicate with each other. Astronauts wear helmets that are part of a sealed system, connected to their spacesuits. Inside these helmets, there’s an atmosphere – usually a mix of oxygen and other gases – which transmits sound. When an astronaut speaks, the sound waves travel through the air inside the helmet, reaching a microphone. This microphone then converts the sound into an electrical signal, which transmits the signal to the communication systems of other astronauts or to mission control on Earth.
Any vibrations caused by an astronaut’s activities on the Moon are felt through their spacesuit. If an astronaut taps on another’s helmet, the latter “hears” it through the vibrations conducted by their spacesuit and helmet.
The Mysterious Music of Apollo
During the Apollo 10 mission, astronauts reported hearing a strange “whistling” sound, which some described as “outer-space-type music,” while they were orbiting the dark side of the Moon. This event remained classified until 2008 and spurred numerous speculations and theories.
However, the source of this “music” wasn’t extraterrestrial. The sounds were likely radio interference between the lunar module and the command module of the spacecraft. When two radios are close to each other and set to similar frequencies, they produce a whistling sound due to interference. This phenomenon, while eerie in the context of space exploration, is quite common and has a straightforward scientific explanation.
Sound on Mars
Mars has a very thin atmosphere composed mainly of carbon dioxide, with traces of nitrogen and argon. This atmosphere is about 100 times less dense than Earth’s. The atmospheric pressure at the Martian surface averages 0.6% of Earth’s sea level pressure. Such a tenuous atmosphere significantly affects the way sound travels on Mars compared to Earth.
Sound travels through the movement of particles in a medium, be it solid, liquid, or gas. The speed and character of sound waves are influenced by the properties of this medium. Given Mars’ thin atmosphere, sound travels slower than it does on Earth. Additionally, the composition of the Martian atmosphere means that certain frequencies, especially higher ones, get absorbed more quickly and do not travel as far.
In practical terms, this means that sounds on Mars are quieter and muffled than we’re used to. High-pitched noises are particularly hard to hear. If you were to have a conversation on Mars without the aid of communication equipment, voices would sound different, and you’d need to be much closer to the source of a sound to hear it clearly.
Are Wind and Dust Storms Silent?
Mars has frequent wind events and massive dust storms. But would a human standing on the Martian surface hear these?
Wind on Mars, even during a strong gust, sounds very faint. Given the thin atmosphere, there simply aren’t enough particles colliding with one another to produce a sound as loud as on Earth.
The massive dust storms that engulf the entire planet are visually impressive, but are surprisingly quiet. The movement of the fine dust and the thin atmosphere does not generate the roaring sounds we associate with storms on Earth. Instead, you might hear a soft hiss or a very low rumble, but it would be much subtler than one might expect.
- Abbott, R.; et al. (29 June 2021). “Observation of Gravitational Waves from Two Neutron Star–Black Hole Coalescences”. The Astrophysical Journal Letters . 915 (1): L5. doi: 10.3847/2041-8213/ac082e
- Everest, F. (2001). The Master Handbook of Acoustics . New York: McGraw-Hill. ISBN 978-0-07-136097-5.
- Kinsler, L.E.; Frey, A.R.; Coppens, A.B.; Sanders, J.V. (2000). Fundamentals of Acoustics (4th ed.). New York: John Wiley & Sons. ISBN 0-471-84789-5.
- Maurice, S.; et al. (2022). “In situ recording of Mars soundscape:. Nature. 605: 653-658. doi: 10.1038/s41586-022-04679-0
Related Posts

Explain why sound cannot travel through vacuum.
Step 1: sound: sound is a form of energy that is made up of vibrations. o bject vibrations cause movement in nearby air molecules. these molecules bump due to these vibrations, and make neighboring molecules excited, causing them also to vibrate. so this process of bumping molecules continues. this bumping causes the creation of sound. step 2: sound can't propagate in a vacuum: sound is a mechanical wave, so to propagate it, some material or medium is required. we know a vacuum is an empty space where no matter particles are present. sound cannot travel through a vacuum as there are no particles present for vibrations to take place. therefore, sound cannot travel through a vacuum..

what is vacuum ? explain why sound cannot travel through vaccum?


IMAGES
VIDEO
COMMENTS
Measuring waves. All sound waves are the same: they travel through a medium by making atoms or molecules shake back and forth. But all sound waves are different too. There are loud sounds and quiet sounds, high-pitched squeaks and low-pitched rumbles, and even two instruments playing exactly the same musical note will produce sound waves that are quite different.
The velocity relation looks like: vsound in fluid = B ρ−−√ (2.1.1) (2.1.1) v s o u n d i n f l u i d = B ρ. Sound will also travel through a solid, but in that case the interactions of the particles are different than in a fluid, and the constant that takes the place of tension is a different one: Young's modulus. But the formula ...
the speed of sound in air is approximately 340 m/s. a high frequency sound wave has a high pitch. large amplitude sound waves are loud. reflected sound is called an echo. the range of human ...
Sound waves can only travel in space if there are enough particles around to transmit the energy in the wave from the source to the listener. If you talk under water, it sounds funny because the water is carrying the sound wave instead of air. Water is a liquid and air is a gas, so water is much denser than air, and the particles are not as ...
A wave is a disturbance that travels or propagates from the place where it was created. Waves transfer energy from one place to another, but they do not necessarily transfer any mass. Light, sound, and waves in the ocean are common examples of waves. Sound and water waves are mechanical waves; meaning, they require a medium to travel through.
Figure 17.3.1 :The mass of a fluid in a volume is equal to the density times the volume, m = ρV = ρAx. The mass flow rate is the time derivative of the mass. Now consider a sound wave moving through a parcel of air. A parcel of air is a small volume of air with imaginary boundaries (Figure 17.3.5 ).
An echo happens when a sound wave hits a hard, ... In a plasma, the physics of sound waves get complicated. Waves travel much faster in this low-density medium, and their wavelength is much longer
The amplitude of a sound wave decreases with distance from its source, because the energy of the wave is spread over a larger and larger area. But some of the energy is also absorbed by objects, such as the eardrum in Figure 14.5, and some of the energy is converted to thermal energy in the air. Figure 14.4 shows a graph of gauge pressure versus distance from the vibrating string.
The stiffer the medium the faster the sound waves will travel through it. This is because in a stiff material, each molecule is more interconnected to the other molecules around it. So any disturbance gets transmitted faster down the line. The other factor that determines the speed of a sound wave is the density of the medium.
Sound can be modeled as a pressure wave by considering the change in pressure from average pressure, ΔP = ΔPmaxsin(kx ∓ ωt + ϕ). Δ P = Δ P max sin ( k x ∓ ω t + ϕ). 17.1. This equation is similar to the periodic wave equations seen in Waves, where ΔP Δ P is the change in pressure, ΔPmax Δ P max is the maximum change in pressure ...
Sound waves are nothing but air vibrations. When these vibrations are in the range of 20 Hz to 20 kHz, we can hear them! Sound waves basically travel by vibrating the particles in a medium, i.e., molecules of air. These vibrations are passed on to consecutive particles in the medium, meaning that sound waves cannot travel without a medium.
Sound waves traveling through a fluid such as air travel as longitudinal waves. Particles of the fluid (i.e., air) vibrate back and forth in the direction that the sound wave is moving. This back-and-forth longitudinal motion creates a pattern of compressions (high pressure regions) and rarefactions (low pressure regions). A detector of pressure at any location in the medium would detect ...
Since sound waves travel at about 340 m/s at room temperature, it will take approximately 0.1 s for a sound to travel the length of a 17 meter room and back, thus causing a reverberation (recall from Lesson 2, t = d/v = (34 m)/(340 m/s) = 0.1 s). This is why reverberations are common in rooms with dimensions of approximately 17 meters or less.
Sound energy can only be perceived by our bodies when it strikes a physical object, like a bone or our skin, causing it to vibrate. This lab will help connect sound production (sources of sound) with sound perception (using our sense of hearing, sight, or touch). Sound travels through space in longitudinal waves.
Sound waves traveling through a fluid such as air travel as longitudinal waves. Particles of the fluid (i.e., air) vibrate back and forth in the direction that the sound wave is moving. This back-and-forth longitudinal motion creates a pattern of compressions (high pressure regions) and rarefactions (low pressure regions).
These are called mechanical waves . Sound waves, water waves, and seismic waves are all types of mechanical waves. Other waves, called electromagnetic waves can travel through a medium or through a vacuum where there is no matter, such as outer space. Light is a form of electromagnetic wave. The amplitude and frequency of both mechanical and ...
Sound waves can travel through any material, but not in a vacuum, which is why there is no sound in outer space. The speed of sound in air is about 330 m/s, meaning that it covers a mile in about five seconds. Sound actually travels at far quicker speeds in other media; for example, in biological tissues, it moves at 1,540 m/s. Cite this Article.
Sound waves are a type of energy that's released when an object vibrates. Those acoustic waves travel from their source through air or another medium, and when they come into contact with our ...
Thus sound waves travel much faster in water than they do in air. In freshwater at room temperature, for example, sound travels about 4.3 times faster than it does in air at the same temperature.
The area in the ocean where sound waves refract up and down is known as the "sound channel." The channeling of sound waves allows sound to travel thousands of miles without the signal losing considerable energy. In fact, hydrophones, or underwater microphones, if placed at the proper depth, can pick up whale songs and manmade noises from many ...
The speed and character of sound waves are influenced by the properties of this medium. Given Mars' thin atmosphere, sound travels slower than it does on Earth. Additionally, the composition of the Martian atmosphere means that certain frequencies, especially higher ones, get absorbed more quickly and do not travel as far.
Reason: Sound waves are longitudinal waves and they cannot be polarised but electromagnetic waves are transverse and they can be polarised. Q. Assertion :Sound waves do not travel through vacuum Reason: Sound waves are mechanical waves which require a medium for their propogation. Q. Assertion :Sound waves do not travels through vacuum.
This bumping causes the creation of sound. Step 2: Sound can't propagate in a vacuum: Sound is a mechanical wave, so to propagate it, some material or medium is required. We know a vacuum is an empty space where no matter particles are present. Sound cannot travel through a vacuum as there are no particles present for vibrations to take place.